Alexander Varshavsky, Biochemist and Geneticist
August 30, September 13, October 1, 7, 19, 26, November 12 and 22, 2021
As its name suggests, the ubiquitin system is present in all eukaryotic organisms. Ubiquitin is a multifunctional regulatory protein that exists either free or covalently joined to many cellular proteins. Among Alex Varshavsky's many discoveries are the first degradation signals in short-lived proteins and fundamental biological functions of the ubiquitin system. These discoveries have proved singularly important for the ongoing efforts, by many scientists, to understand the human genome and intricate designs of biological circuits encoded by the genome.
As Varshavsky recalls in the interview, his early research in the former Soviet Union was stymied by difficulties of doing molecular biology in Russia. He recounts below the thrilling story of his defection to the United States and the beginning of his studies as a faculty member at MIT.
Today, Varshavsky remains most at home in his laboratory, studying the ubiquitin system and other cellular circuits. He joined the Caltech faculty in 1992, initially as Howard Smits Professor of Cell Biology. In 2017, Varshavsky was named the Thomas Hunt Professor of Biology, a professorship in honor of a major pioneer of genetics. A long list of awards recognizing Varshavsky's pathbreaking contributions to biology includes the Gairdner Award in 1999, the Lasker Award in 2000, the Wolf Prize in 2001, the Warburg Prize in 2012, and the Breakthrough Prize in 2014. Varshavsky is a Fellow of the American Academy of Arts and Sciences and a member of the U.S. National Academy of Sciences.
Interview Transcript
[Ed. Note: The first portion of the transcript represents an edited (by Alex Varshavsky) version of eight interview sessions with Varshavsky, interspersed with technical detail provided by him after the original discussion. The second portion of the transcript features Varshavsky's descriptions of his laboratory work and his CV, all provided after the interview sessions had concluded.]
DAVID ZIERLER: This is David Zierler, Director of the Caltech Heritage Project. It is Monday, August 30th, 2021. I am delighted to be here with Professor Alexander Varshavsky. Alex, it's great to be with you. Thank you so much for being with me today.
ALEXANDER VARSHAVSKY: You're most welcome.
ZIERLER: Alex, to start, would you please tell me your title and institutional affiliation?
VARSHAVSKY: The formal title is Thomas Hunt Morgan Professor of Biology at the Division of Biology and Biological Engineering (BBE), California Institute of Technology (Caltech), in Pasadena, California. My lab and I moved to Caltech in 1992, 30 years ago. Until then, my laboratory was at the Massachusetts Institute of Technology (MIT), in Cambridge, Massachusetts. Had transferred the lab to Caltech after working at MIT for 15 years, since 1977. My adventures in the U.S. began in the fall of 1977, less than a month after escaping (defecting) from the former Soviet Union. I was, then, 30 years old. The abrupt move from East to West was made possible by accidents of luck. Some planning was involved as well, but it wouldn't have sufficed without luck. Until 1977, I lived in Russia and worked at the Institute of Molecular Biology (IMB) in Moscow. I joined IMB after graduating, in 1970, from the Chemistry Department of Moscow University.
ZIERLER: Tell me about being named in honor of Thomas Hunt Morgan. What does that mean personally for you, and what does it mean institutionally for Caltech?
VARSHAVSKY: I was invited to work at Caltech as a Smits Professor of Cell Biology. Howard Smits, a kind and gracious man (I met him after moving to Pasadena), gave money to Caltech to establish the Smits "endowed chair". An endowed professorship is an honor, and was good news for another reason as well: my salary was no longer charged to the lab's research grants, as it was at MIT, where I was a "regular" full professor. An institution, understandably, tries to save money. Ergo, there's nearly always a pressure, usually a subtle one, to derive at least a part of professor's salary from his/her grants. This diminishes support available for research. With an endowed chair, my salary was no longer dependent on grants, a welcome change.
The transition from the Smits to the Morgan chair was (unintentionally) funny. A few years ago, I was asked to visit the office of Steve Mayo, then the chairman of Caltech's BBE Division. Ever since my years in Russia, I have been troubled by any request to meet with a boss. Walking to the office of Steve, I tried to recall what I could have possibly done wrong. Steve saw me, smiled and said, "Well, Alex, you're now almost 70 years old". I instantly thought that Steve was planning a scientific symposium in my honor, a jubilee conference or something. What a waste of time such a gathering would be! Before an inkling that I may be wrong had a chance to shut me up, I exclaimed "Oh, no! No jubilee symposium!" Steve was a little annoyed by this silliness and said, "Don't worry about that. There is an endowed chair, probably the most distinguished one in our Division. We would like to suggest that you become Thomas Hunt Morgan Professor of Biology, instead of Smits Professor of Cell Biology. What do you think?" What do I think? No jubilee symposium! A relief! In sum, a little scare, and I became a Morgan professor.
ZIERLER: In terms of who Morgan was, did you ever look into his research or his legacy?
VARSHAVSKY: I knew about Morgan's life and science already in Russia. Before becoming a pioneer of genetics, Thomas Hunt Morgan (1866-1945) has contributed, profoundly, to research on embryogenesis. In 1904, Morgan moved to Columbia University in New York, where his laboratory began to study the fruit fly Drosophila melanogaster. A new model organism in genetics, a branch of biology that was just beginning in those days. Some of Morgan's students at Columbia – Sturtevant, Dobzhansky, Bridges, Muller – became leading geneticists of the 20th century. When Morgan moved his lab, in the late 1920s, from Columbia to the California Institute of Technology, several of his students and former students came with him and founded the great Division of Biology at Caltech.
ZIERLER: Just by way of context, Alex, I'd like to thank you for giving me a tour of your laboratory. It's the perfect prelude for my next question. I know the answer already because I saw the twinkle in your eye when we were in the laboratory. It was so obvious to me what it means to you just to be in that environment. I also know that you recently began working at the bench, doing your own experiments. My question is, why toil at the bench when you don't need to? What's the motivation? Why is it so important for you?
VARSHAVSKY: I'm interested in just about everything, including math and physics. But the brevity of life being what it is compels selectivity. Hence the lab's main subject, the ubiquitin (Ub) system (Figs. 2-5, way below), chosen many years ago, partly through a strong preference and partly by accident.
The arrow of time spares no one, particularly if he or she keeps working beyond what used to be the age of retirement. Until a decade ago, the lab comprised about fifteen colleagues. Now it's five. The reasons are the passage of time and attempts to re-initiate my own work at the bench. Doing experiments by myself requires a small enough lab. Research is about new ideas, designs of experiments, actually doing them, obtaining results worth the trouble, then writing a paper about results, and publishing the paper. By working at the bench, I hope to perform the entire cycle by myself, while still running the lab. A juggling act. Its advantage, if a juggler succeeds, is a decreased dependence on experiments by other colleagues in the lab. A smaller laboratory means a lower productivity. But at this stage of life, a slowdown is hardly a problem. As the late molecular biologist Sydney Brenner remarked, when he was 80, "At my age, the long-term and the short-term are the same thing."
My lab's scientific contributions over the last four decades (Figs. 1-5) are summarized way below, after remembrances and lots of other digressions.
ZIERLER: Doing your own bench work is difficult because there are always conferences to go to, classes to teach?
VARSHAVSKY: One must also write (and preferably receive) research grants that keep the lab afloat. There is, in addition, the running of the lab: thinking about and suggesting projects, assisting colleagues, and writing/publishing papers. Consequently, a majority of faculty members (lab heads) in my profession do not work at the bench, although many of them would love to do their own experiments. After all, it was their bench work, during graduate and postdoctoral years, that led to their faculty positions! But the duties of a lab head and the brevity of a day make an intense bench work impossible for most of my faculty colleagues, with rare exceptions. In my ongoing return to the bench, I did a few experiments and saw how rusty I had become. Both methods and instruments kept evolving over the last 40-50 years.
ZIERLER: What illustrates that? What has changed in the decades?
VARSHAVSKY: For instance, research instruments became more powerful, but often more complex as well. (There are also, of course, instruments that simply didn't exist 40 years ago.) An example, one of many: in decades past, when a biochemist wanted to determine whether two proteins interact with each other, he or she did so by employing methods such as coimmunoprecipitation. Nowadays, you can do analogous assays quantitatively as well, determining the actual affinities of proteins for each other and even the rates ("kinetics") of protein interactions. One instrument for doing this in vitro (in cell-free settings) measures a parameter called "fluorescence polarization". It takes at least a day to learn to use this wonderful tabletop machine and its software. Over the last three decades, several other techniques for detecting protein interactions were introduced as well, including methods for doing this detection in vivo (in living cells). One example is the two-hybrid assay, invented by Stanley Fields and colleagues in 1989 [1]. Nils Johnsson and I contributed to some of these advances, by inventing the split-ubiquitin binding assay in 1994 [2, 3]. Yet another conspicuous change over the last decades was a decrease of sample volumes. Half a century ago, the volumes of samples, in many procedures, were one milliliter or so, but now these volumes are often just a few microliters and even smaller.
ZIERLER: As you say, success at the bench requires long periods of uninterrupted time. If you decide to go emeritus, would that help with your personal work at the bench?
VARSHAVSKY: It wouldn't, unfortunately, since my duties at Caltech, outside research in the lab, are relatively light.
ZIERLER: What about committee work?
VARSHAVSKY: I occasionally serve on committees, including search committees, when my Division of Biology and Biological Engineering tries to identify, worldwide, new faculty members to hire. This work is interesting! You look at new developments in science and meet uncommonly bright young people.
As you know, some years ago, the U.S. Congress essentially forbade universities to compel retirement of attenuated tenured professors, even if they reach shockingly advanced ages. That decision was so beneficial to this particular professor that I try not to think about the U.S. Congress and its collective intelligence. A good idea, actually, given what that august body has become (Mark Twain would say "always was").
ZIERLER: [laughs]
VARSHAVSKY: The lab is still there. But even normal aging is an insidious thing. Recalling my life over the decades, I'm amazed by changes, from one's physical appearance to inner priorities and feelings. Retirement, the cessation of scientific work, is always an option. But what happens, then, to this particular one-trick pony? Cruises, on enormous ships, to exotic places? Watching killer whales being cute at San Diego's Sea World? Writing reviews about advances, by others, in my scientific fields? Penning a memoir? There's nothing wrong about these ways of spending time. The problem is me. Despite attempts to acquire a stronger attraction to daily existence, I can't see a nonscientific life, including friendships, parties and travels, as being even remotely as interesting as doing science.
Reluctance to stop working is common among scientists. Recall Einstein. His last significant scientific paper (with Podolsky and Rosen) was published in 1935, when Einstein was 55. He kept working, largely on unified field theories, during the next 20 years, until his death in 1955. Nearly every day, he walked to the Princeton University from his house on Mercer Street. In his office on campus, Einstein wrote in notebooks or used a blackboard. He often exited the office and strolled about the campus, occasionally entering empty lecture halls, where he wrote, for himself, on the halls' blackboards, then kept walking. In the mid-1940s, someone at the university's administration hired an undergraduate who discreetly followed Einstein, entered a hall that he had just left, and copied whatever the old professor scribbled on a blackboard. A touching story, apparently not apocryphal. It's clear that Einstein wanted to keep working. It is also clear, from the record of his publications, that the results did not even remotely match his discoveries decades earlier, in Zurich, Prague and Berlin.
Health can be iffy even early in life, and becomes more precarious as we age. If health cooperates (it does so far, at 75), I would like not to retire for as long as possible. Would love to play this exacting, unpredictable, and deeply meaningful game to the hilt.
Ann Landers (the pen name of Esther Lederer) was for many years an advice columnist whose answers to readers' questions were a feature in many newspapers. She passed away at 103. When she was 95, Landers was asked how long she intends to work. Her reply: "My favorite dream is to die by falling on my typewriter."
Donald Hall (1928-2018), a poet, was an acquaintance of the writer Philip Roth (1933-2018). Hall described, in his memoir, a conversation with Roth in 1998, when Hall was in Washington. He saw Roth in a line of guests to enter the White House, where Roth was to receive the National Medal of Arts. Roth greeted Hall and asked "What are you doing these days?" Hall: "Well, I'm trying to write." Roth: "What else is there to do?" A version of Ann Landers' reply, and mine.
ZIERLER: Alex, do you serve as your own theorist?
VARSHAVSKY: Yes, largely. Thinking alone is not necessarily the best way, but this propensity goes back as far as I can recall, to my years in Russia, and also at MIT and Caltech. I was born to be a cat that walks by himself. This preference is suppressed, of course, during occasional collaborations with other laboratories, when members of interacting teams, including myself, discuss an ongoing project.
Was your actual question a different one, perhaps? Was it about the extent of separation between theoretical and experimental molecular biology? That distinction is much more fuzzy than the difference between theoretical and experimental physics. Nevertheless, the divergence of theoretical studies from the ones that employ a mix of theory and experiment is already under way in molecular biology and related fields. Some computation-heavy bioinformatics does not involve "wet-bench" studies. If it does, such experiments are often done by other scientists, not by bioinformaticists. There's also, for example, population genetics, which has been largely theoretical (but occasionally experimental as well) since the 1920s. Some areas of neuroscience, such as those that focus on models of neural circuits, are also largely theoretical. Viewed broadly and therefore at a low resolution, this aspect of today's molecular biology is roughly at the stage where physics was at the time of Maxwell in the 19th century. He was one of the first nearly full‑time theoretical physicists, but he also designed and did experiments.
ZIERLER: That's amazing, to think about it.
VARSHAVSKY: Maxwell was a generation younger than Michael Faraday, a great experimental physicist. Faraday knew arithmetic, elementary algebra, geometry and trigonometry. That was about it math-wise. For example, he didn't know (or barely knew) calculus. I have admired Faraday and his science since I was a teenager in Moscow. Read every biography of Faraday I could lay my hands on. He was, first and foremost, a great intuitionist. Did Faraday's under-developed mathematical background stem from the difficult circumstances of his early life? (He was born in a poor family and worked as an apprentice to a bookbinder.) Or was it an innate aversion to a relatively sophisticated math? The latter is suggested (but not proved) by Faraday's largely negative reaction to the original version of Maxwell equations, before the streamlining and other improvements of Maxwell's mathematical notations that were done, years later, by "Maxwellians" (Heaviside, Lodge, Fitzgerald).
ZIERLER: It has been said the last great physicist who never thought about physics in those binary terms was Enrico Fermi, who would never have seen a distinction between experiment and theory.
VARSHAVSKY: True in part, methinks. Fermi was more of a theoretical than experimental physicist, despite having headed an experimental physics lab in Rome and later a group in Chicago, a part of the Manhattan Project, in which he and colleagues constructed and ignited the first atomic reactor. Nevertheless, and in agreement with physicists who know the subject better than me, I think Fermi's greatest contribution to physics was his theory of beta-decay, developed and published in the early 1930s. Arguably his deepest insight.
The late Francis Crick (1916-2004) was one of the first theoretical molecular biologists, if one doesn't count his brief involvement with bench experiments in the early 1960s that used bacteria and phages to explore the genetic code. Crick was tremendously successful as a theoretician in the early years of molecular biology. Recall his coauthorship of the models of DNA double helix and collagen, and his solo ideas about "adaptors" (tRNAs) and the "wobble" mechanism of tRNA-mRNA interactions on the ribosome. In the mid-1970s, when Crick was close to 60, he moved from the U.K. to the Salk Institute in La Jolla, California, and became a theoretical neuroscientist. His contributions to neuroscience over the next three decades were always scholarly and cogent. But all of them, without exception, were not nearly as important for neuroscience as was the impact of his early ideas on molecular biology. Christof Koch's and Crick's work on the nature of consciousness ("neural correlates of consciousness") in the 1990s and later was constructive and good. It finally rescued the subject from philosophers and made things more clear. But not a lot clearer, as we still don't know what exactly consciousness is. I wonder whether Crick regretted, later in life, his decision to change the field of his main interest while remaining a theoretician. People who were close to him may know about this.
My own memories of Francis Crick are warm and full of gratitude. I met him, unexpectedly, in August of 1977, at a conference on chromosomes in Helsinki (Finland), literally on the day I left my life in the USSR for good, by managing to travel from Finland to Sweden without a visa. That transition was helped by Robert Hoffman, an American scientist whom I met during his earlier sojourn in Moscow. The escape adventure, recounted below, was also described in my 2006 interview to Prof. Istvan Hargittai [4] and in a little book by Robert Hoffman entitled, appropriately, "Escape" [5]. When Crick learned, after the Helsinki conference, about my move to the West, he tried to assist me with getting a green card and a job, and was supportive in other ways as well, over many years.
ZIERLER: When you talk about returning to the bench, to what extent was that extra difficult because of the pandemic and not even being able to come into the office?
VARSHAVSKY: I barely noticed the pandemic, since Caltech never closed completely. In fact, I was so lighthearted at first, even before vaccinations, that I didn't wear a mask. Soon thereafter, in the excellent tradition of the former Soviet Union, I was informed upon.
ZIERLER: [laughs]
VARSHAVSKY: Summoned to the carpet, I was remorseful, called myself awful names, and started to wear a mask. Received shots of Moderna vaccine and a booster once they became available. Reading bits and pieces of news on the internet, I felt sorry for people who suffered from the pandemics, but that upheaval was kind of remote, since what I cared most about – studies in the lab – went on largely unabated. Whether Mr. virus will continue to ignore me remains to be seen.
ZIERLER: Do you see any connections between your research and understanding Covid-19?
VARSHAVSKY: My lab does not work on coronaviruses. But our main subjects, the Ub system and regulated protein degradation, play major roles in viral (including coronaviral) life cycles. Inhibitors of a protease encoded by the Covid-19 RNA genome (one of these drugs is the recent Pfizer's inhibitor called Paxlovid) have been shown to be clinically efficacious against coronaviruses.
Modern vaccines cannot encompass the enormous variety of viruses that are capable of infecting humans. In addition, a vaccine is good, in most cases, only before, not after infection, because many viruses cause a disease quite fast. Example: five centuries ago inhabitants of England suffered from epidemics of an illness called sweating sickness. You would know about it if you read Hilary Mantel's Wolf Hall, a first-rate novel set in the time of Henry VIII. Symptoms of sweating sickness usually started in the morning. One felt funny. A fever developed. By the end of the day, the infected person was often dead. Given the swiftness of that disease, it was, most likely, a viral (not bacterial) infection. For reasons unclear, sweating sickness disappeared by the 18th century or even earlier. All this is yet another reminder about the emergency-level importance of developing efficacious, fast-acting antiviral drugs. The ones that exist are already a help, but this field is still at an early stage.
ZIERLER: Is there a grand unified theory of viruses that will allow someday for an all‑inclusive vaccine?
VARSHAVSKY: RNA and DNA viruses emerged as parasites of cells, co-evolving with them, likely at the dawn of a cell-based life, billions of years ago. An antiviral vaccine that encompasses a family of viruses, for example, all human flu viruses, is still a dream but it's likely to be a realistic one. As to an even broader vaccine, the one against literally all (or most) human viruses, it's not feasible in the context of existing vaccines, and would also be unlikely given what we know about viruses. But it's nearly always prudent to avoid the word "never" vis‑à-vis human ingenuity.
ZIERLER: In referring to viruses, do you tend to not think of them as living?
VARSHAVSKY: This is a semantic ambiguity, not a real one. Viruses are not life in the same sense in which cells are. Place a live cell in a non-live setting that contains a sufficient mix of nutrients for a cell to assimilate and use. The cell will grow and divide, producing progeny cells that will do the same. That's life. A virus doesn't fit this definition. If you place a virus particle into a super-rich bouillon that lacks living cells, the added particle won't "multiply", since a virus needs a live (and infectable) cell to start making copies of itself inside that cell. A combination of a virus and a cell infected by it becomes a new "binary" entity in which the virus acts as a replicator, but only for as long as there are susceptible (infectable) cells around.
ZIERLER: Based on what you've seen, do you have any strongly felt opinions about the origins of Covid-19?
VARSHAVSKY: To me, the important issue is not whom to blame. The chief lesson of this pandemic is that it is of paramount importance to develop first-rate antiviral drugs and also to achieve the capability to produce efficacious antiviral vaccines of all kinds on short notice. The latter is already a reality or nearly so, thanks to RNA-based approaches to vaccines, pioneered by Katalin Karikó and Drew Weissman. If strong and rapidly implementable antiviral defenses become widely available, a pandemic like the one we are going through would never happen in the first place.
ZIERLER: On the question of funding, for your lab now and over the course of your career, which have been some of the most important funding agencies for you?
VARSHAVSKY: The taxpayer-supported federal entity called the National Institutes of Health (NIH) remains the chief funder of our lab's research. My two NIH grants support the entire work of the lab. Every four years, a grant has to be renewed through a "competitive renewal" application. And it better be competitive! The application is reviewed by practicing scientists who come to NIH for a couple of days and evaluate grant applications that are submitted by other scientists who work (just as grant reviewers do) at U.S. universities and other institutions all over the country.
Four decades ago, receiving NIH grants was an occasionally difficult undertaking but one with a high probability of success: about 35% (sometimes even 40%) of all submitted applications were actually funded. At that level of competition, it was possible (not easy but possible) to compare individual grant applications in regard to their cogency, originality and the track record of a grant writer.
Alas, comparisons of merit have been going south for years, largely because the number of scientists has been increasing faster than the money that the U.S. Congress allocates to NIH every year. By now, the disposition is awful: only ~12% to ~17% of submitted grant applications (percentages vary from field to field and from year to year) will actually receive funding. That means that ~83% to ~88% of applicants would be informed that they will receive nothing. Not even a fraction of money they applied for.
The difference between 35%-40% (in the late 1970s) and 12%-17% (nowadays) of grants being funded may not seem to be strikingly large. Alas, owing to nonlinear effects, it's actually the difference between a usually fair review and a competition disturbingly close to Russian roulette. With funding cutoffs being what they are today, a single overtly negative remark by a reviewer, even if his or her opinion is incorrect, would often suffice to decrease the "percentile" score of a grant application below a fundable level. The reason, in part, is the rapidity of the reviewing process, as ~20 reviewers have to evaluate, discuss and assign scores to more than 100 grants in two days. In addition, it's impossible, for most reviewers, to be equally familiar with all submitted grants, as each reviewer is saddled with at least six grants to evaluate in detail. In sum, the current funding situation at NIH is a difficult sight. Administrators at NIH and scientists who review grants see the problem first-hand and do everything they can to alleviate it. But when the number of applications and the available dough are so mismatched, there's little one can do.
ZIERLER: Alex, a very broad question. I asked above about observation and theory. Another binary – your approach to basic science versus applied science, or even medical therapies. How do you think of these things in terms of defining your research, understanding your motivations, and setting up the lab work? Where in your world is there the basic science, just understanding how nature works, and when do you have goals in mind for what you want to apply the research toward?
VARSHAVSKY: A character played by Clint Eastwood correctly pointed out that "a man's got to know his limitations". In my youth I felt that days are long, life is nearly infinite, and a strong motivation can move a mountain. A delusion of this kind is dangerous but can be helpful too, since you behave like a fearless drunk who can tackle things that sober people would not even think of dealing with. As an undergraduate at the Moscow University, I thought I would discover a curative therapy for cancer in short order. After that, I would deal with the problem of aging, and would solve it too, of course. Around that time, I read a remark by Ludwig Boltzmann about Gustav Kirchhoff (1824-1887), an outstanding German physicist two decades older than Boltzmann: "There were no great events in his life. Great events took place in his head." I liked to think that a latter-day Boltzmann would write something like that about old Varshavsky. This interesting psychological state left me (well, almost left) by the time I was 30.
Psychiatrists and other observers of human minds pointed out that having a fire smoldering in you, having a low-intensity mania grandiosa (below levels that qualify one for a mental asylum) creates a phenotype that is bolder and braver than it would have been otherwise. A state like that, despite its downsides (such people tend to be odd and difficult, to themselves as well), can be helpful in professions that thrive on exceptional motivation. You may not be deranged enough to believe that you are another Newton or Euler, or Einstein, or Feynman. But it's helpful to forget about this inconvenient fact and to actually see yourself, in a cozy little delusion, a bit closer to these people than you actually are. I felt, over the years, that there's no such thing as too much motivation. Provided that your love of science, a separate trait, is in place as well.
ZIERLER: The motivations for what? For understanding or for applying the understanding?
VARSHAVSKY: The word "ambition" signifies different things in different arenas. A politician wants power, influence, dominance. If voters are lucky, he or she would also not object to governing well. The ambition of a researcher is different. The definition I particularly like is the desire to be a better scientist than one's colleagues, living or dead, whose work one admires. If you plan to be an experimental physicist, would you like to be a better one than Faraday? You probably would, despite the near impossibility of it. The above dichotomy between politicians and scientists is not as neat as it looks, because human nature is protean. Some (not many) scientists are power seekers and sleazebags. Some (rare) politicians are a sight to behold: decent people in their profession, which is nearly as far from scrupulous as any profession can be.
About applied versus basic science: during early undergraduate years I was thinking about curing cancer, with (expectedly, in hindsight) negligible results. Later, I saw that one has to be extensively, deeply informed, while trying to glimpse a helpful idea. Some people tend to have genuinely new thoughts not infrequently. Most of these ideas are wrong or "not even wrong" (totally astray), but once in a while, a new thought is not only correct but also solves (or nearly solves) a significant problem. New ideas came to me often, and continue to arrive even now. Most of them are barely realistic or belong to research arenas too far from my immediate interests. I keep writing down some of those thoughts, without a hope of implementing them, at the young age of 75. In these and other attempts to discern what to tackle next and how to do research, the applied and basic biology are both separate and together, like different toys in Santa Claus's bag.
My chief interest, throughout life, was in solving molecular biological problems, the more fundamental the better, and also in inventing new methods. Major inventions in molecular biology, for example polymerase chain reaction (PCR) [6], two-hybrid assay [1], and the approach to gene editing called CRISPR-Cas [7], illustrate the long-ago understood truth that an invention of a first-rate method is, in a sense, more important than a specific discovery, since a powerful new technique enables many discoveries, often in short order.
In 1995, 1998 and 2007 I published theoretical papers that proposed ideas about small compounds [8] and molecular biological (DNA/protein) constructs [9, 10] with previously unattainable properties. Later, in 2012 and 2019, I proposed verifiable ideas, summed up as the fragment generation (FG) hypothesis, about a possible molecular cause of sleep [11, 12]. The FG hypothesis is described way below.
A priori, it would be great to have a small-compound drug that would selectively bind to (and, for example, inhibit) a cellular target, e.g., a specific protein, while doing it in a way that could be modulated by other specific proteins in a cell. The 1998 paper proposed a design for such compounds [8]. But my lab did not try to implement it, in part because I'm not a practicing organic chemist, and also because I came to think that it would be difficult to develop a pharmacologically realistic drug based on designs in that 1998 paper [8]. Whether a version of these designs may eventually prove helpful, I know not.
The 1995 paper introduced the concept of a short-lived protein effector whose stability can be modulated by specific cellular proteins that would bind to two or more degradation signals (degrons) in the effector protein and conditionally increase its half-life (and therefore its relative activity) by shielding the effector protein from attacks by the Ub system [9]. Since multiple (and different) degrons of the effector protein would be shielded independently of one another, this design suggested, on paper so far, the possibility of protein-based drugs that could be "regulated" by more than one cellular protein. A modulation of a specific degron by proteins that shield that degron from recognition was subsequently demonstrated by us and others. But a set of designs proposed in [9] remains to be implemented.
The 2007 paper [10] described an approach to cancer therapy that involves homozygous deletions (HDs) of unique (nonrepetitive) DNA sequences. A homozygous deletion, caused by genomic instabilities of most cancers, differs from other attributes of a cancer cell by the fact that an HD cannot revert. Thus, a treatment that targets exclusively cells that lack specific DNA sequences that are present in normal cells may be not only curative but largely free of side effects as well. The difficulty here is that an HD is the "absence", i.e., it cannot be a conventional molecular target. Nevertheless, an HD-specific regimen, termed deletion-specific targeting (DST), is feasible, on paper so far, at the price of complex DNA/protein-based designs [10]. The notion that underlies and motivated DST [10] is that a consistently curative therapy may require polymer-scale, multitarget, Boolean-type molecular circuits, i.e., that simpler (smaller) drugs might not suffice, particularly with regard to side effects. The task at hand is to address the validity of this assumption.
ZIERLER: Did your interest in science develop early?
VARSHAVSKY: Having escaped, at 30, from the professional constraints of working in Russia, I sensed my total commitment to the craft, which I did not perceive as a profession at all, but rather as the only thing I would ever wish to do, the only thing worth spending life on. Such a feeling, impossible to acquire at will, makes one much less vulnerable to the usual career concerns. The attraction to science started early, during my high school years, in part because my father was a scientist. His initial studies, after WWII, aimed to develop methods for industrial-scale production of heavy water (D2O). That work, a blend of basic and applied physical chemistry, was a part of Russia's atomic bomb project. In 1953, my father came across the two‑page paper by Watson and Crick about the DNA double helix. He started to read papers about DNA and became interested in biological studies! By the late 1950s, he joined other Soviet physicists and chemists who were leaving their fields for the nascent world of molecular biology.
One day, in 1969 (I was an undergraduate student), my father came home from his laboratory at the Institute of Molecular Biology (IMB) in a palpably bad mood. He said, "A U.S. scientist, named John Shell, was visiting IMB and came to my lab today. Well, I did what I usually do on such occasions. I complained."
ZIERLER: [laughs]
VARSHAVSKY: "I said that reagents are dirty, there is no money, the ruble is worthless outside the Soviet Union, we cannot do this or that, we cannot buy any decent equipment. Having finished that spiel, I looked at the lucky American professor, but his face expressed nothing. Not a flicker of sympathy. He was also silent. What do I do? I double my complaints."
ZIERLER: [laughs]
VARSHAVSKY: "This is lousy. That is lousy. The situation is impossible and unbearable. And so forth. Not a sound from Dr. Shell. I was about to give up, when he finally opened his mouth, and said, "You know, Professor Varshavsky, I envy you. You have an excuse."
ZIERLER: [laughs] That's great!
VARSHAVSKY: I remember that evening, five decades ago, as if it happened yesterday. I thought, then, that I want to work at a place where excuses don't apply, where I wouldn't be able to blame anything or anyone but myself for professional failures, if such were to occur. My attempting, later, to move to the West was propelled, in part, by the desire to find such a place. And I did, less than a month after escaping from the USSR, when colleagues at MIT's Biology Department offered me a position as (independent) assistant professor. Fortunately, they hired me before they realized how ignorant and uninformed I was about the logistics of doing science in the U.S. For starters, I didn't even know what grants were or how to apply for them.
Returning, after all these tangents, to your earlier question, a short answer is that distinctions between applied and basic science are fairly well defined, but I barely think about these differences. I would like, very much, to do something interesting. If a significant advance happens to be in the domain of applied biology, wonderful. If it's good for the human condition, I would be happy. But "interesting", i.e., unobvious, original, and also, if possible, helpful, is the keyword.
ZIERLER: Can you be a scientist in Russia today and not have excuses?
VARSHAVSKY: Yes, if you are a mathematician or theoretical physicist. That was true even before the 1991 collapse of the Soviet Union. Leading mathematicians in Russia were second to none at that time, and theoretical physics was world-class as well. But the situation with, for example, experimental molecular biology was quite bad in the 1970s (the years when I worked in Russia), largely because first-rate experimental science depends on having first-rate material support: readily available scientific instruments and pure enough reagents.
(This paragraph was written before Feb. 24, 2022.) The state of molecular biology in today's Russia is better than it was 50 years ago. But not qualitatively better. First-rate studies that have been performed entirely in Russia remain a rare sight in the leading journals of our profession. A significant reason for this is different from the old reason of material poverty and isolation behind the Iron Curtain. The main current cause is the brain drain. After the demise of the Soviet Union in the early 1990s, the possibility of leaving Russia legally was embraced by many of the brightest and best among young (and not so young) Russian scientists. They no longer depended, as I did in 1977, on the rare luck of being able to escape from the country whose citizens lived, in effect, as inmates in a prison. Owing to a massive (and still continuing) exodus of Russian scientists that began three decades ago, there are, quite often, Russian‑sounding names on excellent, insightful papers from Western universities and pharmaceutical/biotechnological companies in which these emigrants from Russia work nowadays.
I'm editing this section of the narrative further in early 2022, five weeks after the Russian army invaded Ukraine and started Putin's criminal war. The recent, massive and justified sanctions by the (still) free world will damage not only the Russian war machine and economy at large but also, inevitably, the ability of Russia to support fundamental science. At present, the only possibility for a young scientist in Russia to carry out original and extensive studies in molecular biology would be to do that work outside the country, if he or she can, since the meager support of basic experimental research in Russia will continue to decrease.
Igor Guberman, a poet and writer, left Russia decades ago. Quite a few of his rhymed bon mots are hilarious, insightful and poignant at the same time. A recent four-liner by Guberman, shown here together with a photo of its author, doesn't mention Putin by name but it's clearly about him and characters like him. The translation, below, from the rhymed Russian is not rhymed, alas:
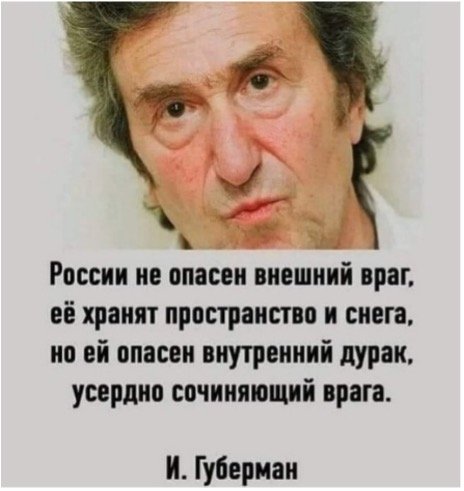
ZIERLER: Alex, I'd like to ask about your overall approach to science, that even has philosophical implications, and that's a topic that you've written about, where you tend to deemphasize the distinctions, the human constructs where we put biology in this column and chemistry here, and physics here.
VARSHAVSKY: Ah, you probably read that little disquisition I wrote in 2008, entitled "The World as Physics, Mathematics, and Nothing Else" [13].
It would be good if more people outside of science would see it as a unitary enterprise. Among practicing scientists, it's a given that science is unitary. When we talk about psychology, or linguistics, or high-energy physics, or chemical reactions, or living cells, we are talking about different aspects of the same subject. The names of different sciences, be it "chemistry," "astronomy," "immunology," "linguistics," "psychology" and so forth, are shorthands that indicate the location of a specific domain of science vis-á-vis its other domains. In part because of multiplicity of these names, the unitary nature and hierarchical structure of science tend to be less obvious to nonscientists.
In that 2008 piece, which you saw, I proposed to make the terms "science" and "physics" synonymous. There was also a suggestion about philosophy and its discontents. In what follows, I'll cite a few excerpts from that paper [13].
The statement that chemistry has been reduced to physics is correct in the well-defined sense of interactions that involve small numbers of atoms. It is most likely that this statement is also correct in principle, for arbitrarily large molecules, their large ensembles, and their reactions. This reductionism "in principle," as distinguished from "practice," underlies physics proper as well. The "arrows of explanation" are far from random: they point, consistently, from complex systems, e.g., those encountered in condensed-matter physics, to systems with fewer and smaller components. These arrows eventually converge on the level of quantum mechanics of elementary particles and atomic nuclei composed from them [13].
In sum, what I suggested was not a conjecture but a set of definitions that have (as they must) the property of self-consistency but aspire to nothing greater than that [13]. Specifically, let us call "physics" what is traditionally denoted as "science." "Science," in that context, would be simply a synonym for "physics." This definition addresses, in one step, the often-encountered confusion, among nonscientists, about the multiplicity of "sciences," as if they are separate entities. They are not, and the above definition takes care of that [13].
Physics proper, then, would be defined as the set of domains (high-energy physics, astronomy/cosmology, condensed-matter physics, applied physics, etc.) that are conventionally denoted as "physics." A particular subset of physics, called "chemistry," is defined as physical systems that involve large sets of atoms significantly above 0°K (absolute zero) but at sufficiently low temperatures and pressures to enable reversible interactions and formation of metastable atomic aggregates called molecules. That's chemistry.
Biology, then (at least biology known to us), is a specific subset of chemistry (and hence of physics) that involves temperatures between ~0°C and ~200°C, plenty of H2O, carbon/nitrogen/oxygen/phosphorus-based polymers and a multitude of small compounds that come together, in highly (but finitely) complex ways, and form living cells. Such cells have the properties of replicators, i.e., the ability to assimilate parts of media in which a replicator grows and to make copies of itself. The imperfection of copying results in mutations and leads to a variation in the frequency of survival of replicator's progeny, thereby making possible evolution of cells and multicellular organisms through "natural" selection, i.e., through a competition among nonidentical replicators.
If science is physics, what about philosophy? Richard Feynman's disdain for the subject would be obvious to anyone who read his Lectures on Physics: "These philosophers are always with us, struggling in the periphery to try to tell us something, but they never really understand the subtleties and depths of the problem." [14]. The beginnings of philosophy, in ancient Greece and earlier, were synonymous with the emergence of science. Later, much later, subsets of science that had defined themselves through experiments and mathematics were escaping, in droves and for good, from philosophy's vagueness and high-falutin discourses that often covered up the absence of content. A succession of parades, throughout the 20th century, by obvious charlatans like Marxist philosophers in the former Soviet Union, the "intellectuals" like the late Jacques Derrida in France, or philosophers like him in other places, did nothing to increase respect for the subject among scientists who bothered to take a look at the writings involved.
It's odd that the deepest questions (for example, the nature of causality, the nature of spacetime and its quantum aspects) are kind of partitioned, in an ill-defined way, between physics and philosophy. The former is one of humanity's greatest achievements: it led the way, hand in hand with mathematics, in our learning of how to think more accurately, so that we don't end up fooling ourselves the moment a question is even halfway subtle. In contrast, the field of philosophy, once hard sciences separated from it, became a depressing sight.
"If your horse dies, we suggest you dismount." [15]. This, in a nutshell, is what I propose to do. It's time to see that philosophy was a transient, scaffold-like enterprise, with a beginning and an end. The latter could already be glimpsed in the 19th century. The reformulation, below, is constructive, in that it doesn't "cancel" anything but relegates all subjects of philosophy to specific branches of science. These branches, as we already saw, can be defined (not proven to be, but defined) as subsets of physics.
The proposed reformulation can be stated in operational terms: suppose that one is presented with a philosophical statement that claims to have a specific, verifiable-in-principle content. Suppose, furthermore, that a close examination of that statement confirms that it is, indeed, likely to have "content". If so, the reformulation predicts that this philosophical statement can always be classed as actually belonging to science. For example, a "content-positive" statement about the nature of mathematical proofs, or about human motivation, or about human moral dilemmas, or about spacetime can be viewed, without a stretch, as a statement in mathematics, in neuroscience, in psychology (a branch of neuroscience), and in fundamental physics, respectively. Whether such assignments are always possible, and whether, as a result, philosophy departments at universities are based on a long-term, unfortunate misunderstanding, remains to be seen [13].
ZIERLER: Alex, we discussed before I started recording, how you see distinctions in science between "mysteries" and "puzzles." What are those distinctions?
VARSHAVSKY: A puzzle is a problem that has not been solved but appears to be tractable, i.e., one can think of approaches that might be able to solve it. A mystery is an intractable problem. So intractable that there's a seeming impossibility (or at least a great difficulty) of guessing where to even begin. Distinctions between mysteries and puzzles are not carved in stone. Two millennia ago, even uncommonly thoughtful people saw the world as being full of mysteries. Over the last four centuries, the immense, incredible, astounding advances through science (and its brainchild, technology) tackled most mysteries either by solving them or by converting them to puzzles. At present, mysteries are confined to just one domain: a subset of physics that encompasses the origin and nature of the universe at large, the nature of spacetime and vacuum, their quantum and large-scale aspects, and analogous fundamental problems. Religious folks would disagree about that domain being the sole location of mysteries. Over the last decades, Richard Dawkins, Steven Weinberg, and many others tried to explain things to the religiously inclined, but a majority of people (a decreasing majority, though) continue to see the world through the eyes of organized religions.
ZIERLER: Speaking of mysteries, let's consider cancer. What about cancer is a mystery, and what's a puzzle?
VARSHAVSKY: Cancer is a large set of related but distinct and mechanistically diverse illnesses. Cancer (more accurately, cancers) is no longer a mystery. Owing to major advances in cancer research over the last 50 years, we understand the molecular nature and origins of specific cancers quite well, and the understanding keeps becoming more detailed. As to a cure for cancers, it has been achieved, so far, to a significant (but not 100%) extent for some (only some, alas) of these diseases. Cancers are caused by mutations (some of them, not all of them) in one's DNA that occur throughout the body during one's lifetime (including mutations incurred by a fetus during its growth in the uterus), and also by effects of germline mutations inherited from parents. I'm almost certain that curative and (nearly) side effects-free therapies of most cancers, based on their detailed understanding, are a realistic aim. Most cancers will be cured, comprehensively and reliably. Roughly a generation from now, I hope.
ZIERLER: There are no mysteries in biology at this point?
VARSHAVSKY: None that I know of. But puzzles, including difficult puzzles, abound. Even the detailed nature of consciousness is a challenging puzzle but not a mystery, methinks.
That said, "mysteries" and "puzzles" are not more than labels, reflecting one's bias. The relevant thing is to advance the understanding. The rest would take care of itself.
ZIERLER: I'll ask a simple question that will lead to a complex question. I know you're not a religious person, but I don't know if you're agnostic or atheistic. Are you sure there's no God, or are you willing to hedge?
VARSHAVSKY: As a historian of physics, you would be the first to agree that the question is poorly formulated. I don't know what God is.
ZIERLER: But religion gives you a very good idea of what God is.
VARSHAVSKY: I beg to differ. Concepts of God are so fuzzy (let alone varying) and so utterly unfalsifiable that the entire idea does not qualify as a concept, in my book. In addition and independently, there is also a problem that is obvious to a logic-respecting person once the notion of God is broached. Let's forget, for a moment, about imprecisions of God's definitions. If there is God, the one who created reality (universe, whatever), who, then, created that God? And who created the third-order God who created the second-order God who created the first‑order God, the one that is initially invoked? This problem alone indicates that the notion of God is ambiguous at best.
I am neither an agnostic nor atheist. The latter term is often used to describe a combative stance that denies the existence of deities. My case is anything but combative. I am nonreligious and don't think highly of thought processes that lead to a religion. First, for the reason of a poor definition mentioned above. In the vast variety of extinct and extant religions, a God (or Gods) is stated to be a supernatural entity, in the absence of genuine (let alone successful) attempts to define, in a logically acceptable manner, what "supernatural" is. Second, the notion of God is not falsifiable, even if there is no punishment for doubting the concept, like being burned at a stake, courtesy of the Inquisition. The third and also important reason: the notion of God is unnecessary.
In regard to the third reason, there is a possibly apocryphal (but telling anyway) story about Napoleon Bonaparte and Pierre Simon, marquis de Laplace, a great mathematician and physicist. For a tyrant, Napoleon was well educated. For example, he knew some calculus, a requirement for an artillery officer he was in his youth. Having encountered Laplace at a ball in a palace, Napoleon was said to remark: "They tell me you have written a large book on the system of the universe, and have never even once mentioned its creator". Laplace's reply: "Sir, I had no need of that hypothesis."
Religions likely co-evolved with language, becoming more elaborate over the last ~100,000 years, as human vocabularies and sophistication of syntax gradually increased. Specific reasons for the emergence of religions included their adaptive (survival-enhancing) quality, a solace and comfort in the frightening and cruel world. As time went by, religions began to be used as instruments of power. Organized religions held sway for millennia but became untenable with the rise of modern science. Nevertheless, churches, temples and mosques continue to exist, and religious individuals are a majority in the world. But a dwindling majority, owing to global increases of literacy and education, and also, critically, due to modern science.
My mother told me that that her ancestry is traceable to the chief rabbi of Prague in the early 17th century. Had I been born in the Jewish ghetto of a semi-medieval Prague, I might have ended up a religious scholar, splitting hairs with fellas at a yeshiva about subtleties of Talmud and Torah. Although it's agreeable to hope that I would have discerned, unaided, the incorrectness of a religious outlook, this would be quite unlikely. It is difficult, nowadays, to appreciate the acuteness of insight and independence of spirit that would be essential for such a discovery before the rise of modern science.
Nevertheless, rare individuals, both in antiquity and in the Middle Ages must have glimpsed this insight. That the names of early doubters are largely unknown to us is no accident. From times immemorial to roughly the 1700s in Europe, one could safely declare a disbelief in deities and their deeds only in total solitude. Even centuries later, the nonbeliever's view of extant religions is a majority opinion only among scientists (particularly hard scientists), but not yet in the public at large.
Many centuries ago, rare nonreligious individuals could not rely on a still rudimentary science to surmise that a religious view of the world is likely to be incorrect. What was the source of their perspicacity, in the absence of understanding provided by science? In my view (can't prove it, of course), their insight required an uncommonly robust common sense. Not the entire common sense but a subset of it, the one that depends on what is called brights, the innate discerning intelligence.
Oversimplifying and using a decidedly nonscientific term, the requisite quality involves a particularly well-developed bullshit detector. Versions of this detector are present in all of us. Interestingly, however, the detector's sensitivity setting is on "low" in many people, which is why charlatans of various kinds – televangelists, homeopaths, a majority of politicians – continue to be successful.
The long-term trend, on the scale of centuries, although a turbulent one, with transient local reverses, is clearly toward a secular, science-informed outlook. A stance in which phenomena we don't have good explanations for are called mysteries (currently intractable problems) or puzzles (tractable problems), in the absence of attempts to camouflage an insufficient understanding by theology and fairy tales. It has been remarked by others that a distinct mark of intelligence is unwillingness to fill gaps in one's understanding with irrational beliefs.
ZIERLER: Alex, now we are going to shift to your life. In our first session, we got the wonderful sense of your approach to science, and your command of the history of science, and where you see yourself and your career within that larger context. A question to get things started: what were your family's experiences during World War II?
VARSHAVSKY: Some (only some) parts of the narrative below are reworded versions of segments from the previous interview, of 2006, with Prof. Istvan Hargittai [4].
My mother Mary Zeitlin and father Jacob (Yakov) Varshavsky were acquainted before WWII, and got married in 1942. My mother's family was fortunate to be evacuated in time from Kharkiv, a city in Ukraine that was overrun by the Wehrmacht in the blitzkrieg offensive of 1941. (Note added on April 7, 2022: for more than a month by now, both Kharkiv and other Ukrainian cities are, yet again, the scenes of unspeakably cruel and massive bombardments, this time by the Russian army, which claims to "liberate" Ukrainians (while killing them) from a concocted enemy that no one can see.)
Most of those Ukrainian Jews (including women and children) who did not manage to be evacuated to eastern regions of the USSR in 1941, were rounded up by SS battalions and summarily shot, long before the concentration camps and gas chambers of the Final Solution. My father graduated from the Chemistry Department of Moscow University two years before the war. He was drafted in 1941, joining a tank battalion of the retreating Soviet army, and was injured soon afterwards. A piece of shrapnel hit his kidney, and chronic damage ensued. He was never sent to the front again. That injury, bad as it was, was a stroke of luck, since few returned home among those who began as soldiers in 1941. Father's older brother Isaac was a military officer on a submarine based in Sevastopol, on the Black Sea. He was in combat from the start of war, and died two years later, in 1943, on a destroyer that was bringing him to Sevastopol's base and was sunk by a German submarine.
ZIERLER: What are your first memories?
VARSHAVSKY: My father, mother, I, my sister Marina (born in 1954, when I was 7), and our nanny Maria ("Marusya") lived in a single room of a communal apartment in downtown Moscow. The apartment was typical for its time, in the city desperately short of decent housing. It was a corridor with fifteen doors, which led to single rooms. Each of them housed a separate family.
One of my earliest recollections, at about 5, were bottles of milk. Thanks to my father's work with D2O (heavy water, a part of the atomic bomb project), he was given "free" bottles of milk, on the account of his working with "isotopes". Deuterium is a stable isotope, but never mind. The difference between stable and radioactive isotopes was too much of a subtlety for officials who shepherded scientists in their employ. Instead of drinking milk at the institute, my father brought it home. I was told that the milk was from mad cows, a frightening but intriguing description to a 5-year old, many years before "mad cow" came to signify a less benign proposition.
An incident a year later: I was about 6, and didn't know I was Jewish. Also didn't know about nationalities. No one explained them to me. I spent most days with other small kids, looking for ways to kill time in the yard of our apartment house. "Zhid" is the Russian counterpart of English "kike", a derogatory term denoting a Jew. There was a Jewish boy named Michael in the yard. He was about my age, a timid little fellow who wanted to be a friend of everyone. Slightly older boys (mostly boys) who knew what "zhid" meant and also knew it was safe to taunt a small enough kid, kept shouting "zhid", "zhid" and pointing fingers at Michael. He was so timorous that he simply stood there, instead of returning to his apartment. I was caught in the crowd's excitement and also shouted "zhid" at Michael. My father was returning from work. He saw the spectacle and my great performance. Most of his anger was toward his son. He dragged me to the apartment and beat me with the belt of his pants. Calming himself afterward, he told me that I am a Jew, that "zhid" is a bad word denoting the same, and that there is nothing wrong about being a Jew. On that lovely day, my education about nations was off to a good start.
ZIERLER: Not to play psychologist, but I wonder if part of your father's reaction was disappointment in himself for not educating you on your traditions and your background.
VARSHAVSKY: He was upset probably not as much by what I was shouting as by the fact that he had a stupid and cruel son who may grow up a stupid adult. Even if I didn't know what "zhid" meant, it should not have required a lot of insight to avoid joining a crowd of boys taunting a smaller one.
My parents were assimilated nonreligious Jews. They couldn't possibly forget about being Jewish, since reminders never ceased. Post-WWII antisemitic campaigns instigated by Stalin and his government included dismissals of Jews from their jobs and often calamities worse than that. These campaigns came to a halt soon after the death of Stalin in 1953.
Time flew. By the late 1950s, my family moved to a small but separate two-room apartment. Friends of my parents often visited them. Having become a teenager, I began to see scientists among my parents' guests as particularly interesting people. A din of conversations about physics, chemistry and biology surrounded me at dinner parties, with serious talk dwarfed by jokes, laughter, and political commentaries that would have been unthinkable in Stalin's time. The regime didn't lose its fangs but the willingness to use them had diminished, and people were emboldened a bit.
By 16, I wanted to do all of science, mathematics included, dreamt of becoming a writer too, and felt, without evidence, that all of this was possible. That mania grandiosa eventually subsided, perhaps not entirely. To the world outside I was a typical "academichesky malchick", a Russian idiom for "professor's son": an alloy of cockiness, nerdiness and insecurity, the latter camouflaged by arrogance. To myself, I was the eighth wonder of the world. A secret knowledge, but it surfaced with regularity that must have made dealings with me a chore.
My initial interest in science soon upgraded itself to love. Memoirs by scientists, their biographies, particularly of physicists and mathematicians, became my favorite reading, and the personages themselves my closest friends, all the more so because I was ill at ease with real people. At about 17, I read Einstein's remark that one cause of his attraction to science was the desire to be shielded from everyday existence, from its unbearable cruelty and inconsolable emptiness, from the prison of one's constantly changing whims. I was astonished he felt that way, for I did too, but didn't discuss the subject with anyone, then.
In 1964, when I was about to graduate from high school, my father told me approximately this: "So you are interested in biology. Good. But ignore biology for now, kind of. Get the best background in math, physics and chemistry that you can possibly achieve, then worry about biology. Learning biology is much easier than physics and math, so focus on them first." By then, I knew enough to immediately sense he was right.
ZIERLER: Did your early education emphasize Communist ideology?
VARSHAVSKY: "Emphasize" is an understatement. My parents were afraid to share, even indirectly, their political views with their talkative progeny. A cherubic kid may be innocent enough to chat at school about mom's and dad's conversations at home, with consequences for the entire family. I had to understand how things were largely by myself. It began to happen by about 13, as I started to notice inconsistences in the official propaganda. Its main declaration was that we, the Soviet people, were the luckiest people on earth.
But between ~7 and ~11, I saw propaganda as a given truth. We were told, at lectures about future life under Communism, that everyone will have more than enough and no one would have to do much. We liked that arrangement a lot! I felt sorry for children in capitalist countries, who were exploited, beaten, and hungry. Somewhere between 13 and 14, most of that stuff was gradually replaced by understanding based on facts.
ZIERLER: Alex, did the 1962 Cuban Missile crisis register for you?
VARSHAVSKY: I was nearly 16 in 1962, still felt immortal, and was surprised by the concern of my parents and their friends. My father thought that the aggressive and idiotic gamble of Khrushchev and Castro would be likely to result in the utter destruction of Moscow by an atomic or thermonuclear bomb. Some among my parents' acquaintances fled from Moscow during those weeks, moving to villages far away from the city.
ZIERLER: What led to your decision to become a student at Moscow University?
VARSHAVSKY: A "decision" to be a student at the Moscow State University (its Russian acronym is MGU (МГУ)) was necessary but far from sufficient for actually becoming one. The competition to enter specific departments of that flagship institution was so high that the entrance exams (at least in the 1960s; I don't know about now) were held a month before exams at other educational institutes, so that applicants not admitted to MGU could try their luck at other places as well. I applied to the Chemistry Department of MGU in 1964, having decided to do biology but also planning to learn chemistry, physics and math. There were several entrance exams (math, physics, chemistry, and "literature"). I received "A" in all of them, and was admitted.
During the second year of studies at MGU, I began moonlighting in a biochemical lab, and loved that work. At nineteen, in 1967, listening to a lecture on quantum mechanics, I was visited by the first genuinely new scientific idea I ever had. Around that time, DNA-binding genetic repressors, predicted by Jacob and Monod in the early 1960s, were demonstrated (by Gilbert, Müller-Hill and Ptashne) to actually exist. But the thought I had was different: what regulates repressors? Could it be that repressors regulated themselves, for example by inhibiting the synthesis of their own mRNAs? (mRNAs had been discovered in the early 1960s.) I was so stunned that such a simple idea might be new, let alone correct, that I bolted from the classroom, went to the library and buried myself there, forgetting such trivia as attending lectures and laboratory courses. Skipping lectures was not a lethal mistake, as one could prepare for exams by reading textbooks. But playing hooky from practical courses was madness, since one had to complete them to qualify for the next semester. Predictably, I came very close to expulsion from the university. That would have been a disaster (of my own making), for I was, by then, of sufficient age to be immediately drafted for a three-year stint in the Soviet Army.
The reasons I wasn't expelled from MGU were my having been a straight-A student up to the moment of thinking up the repressor idea, and also an accident of fate: my father and Lutsenko, the chairman of MGU's Chemistry Department, knew each other, as both of them were MGU's students shortly before WWII. At Lutsenko's request, my father and I came to the chairman's office. The moment he saw us, Lutsenko said: "Yakov, do you know that your son is an idiot?". "All too well", answered my parent. It was mostly Lutsenko's kindness and his memories of camaraderie with my father that led to the decision to allow me to remain a student and to re‑enter practical courses that I had blithely ignored.
Thus rescued from the deserved punishment, I kept reading scientific journals, prepared a theoretical paper, and furnished it with differential equations that described the behavior of molecular circuits in which repressors regulated themselves. The paper was published in the January 1968 issue of Russian "Molecular Biology", then a new journal [16]. Nowadays, the modeling exercises I labored over in that paper would be called "systems biology". My version of it was too simplistic to be of any use. But the idea itself was new and turned out to be correct for a number of transcriptional repressors. Models of biological regulators regulating themselves began to be considered in English-language papers around 1971, naturally without reference to the 1968 paper [16] in a relatively obscure Russian journal.
That idea, and my coming close to expulsion from university because of it, were a searing experience. I saw that I was capable of thoughts that were new, and possibly even correct. I also saw, a bit later, that my penchant for equations should be postponed for a remote future, since specific regulatory systems at hand were too dimly understood in the biochemistry of the early 1970s to allow quantitative modeling realistic enough to be useful.
ZIERLER: What was the state of molecular biological studies in the Soviet Union during the 1960s-1970s?
VARSHAVSKY: The shadow of Trofim Lysenko was still visible in the 1960s. He was a textbook example of a power-hungry demagogue and charlatan. A wily intriguer but also a limited man who posed as a scientist (and probably saw himself as such) but never understood science. In the mid-1930s, Lysenko began his campaign against the small community of Soviet geneticists. His power was based on his support from Stalin, to whom Lysenko promised (but never delivered) great agricultural harvests and cows producing rivers of milk. Stalin and Lysenko had much in common as sociopathic characters and chieftains, but differed in their demeanor, with Stalin reserved (in public) and Lysenko a histrionic bully. Stalin held the entire country in his fist, while Lysenko's fiefs were biology and agriculture. By the late 1940s, Lysenko became the tsar of whatever remained of Soviet biology. Several leading geneticists were killed or imprisoned. The lucky ones were fired, or dropped their studies early enough and turned to safer occupations. Lysenko's own brand of "Marxist" biology is not worth recounting. There are books in English about that Rasputin-like figure.
One consequence of Lysenko's reign in Soviet biology was the near disappearance of people who understood genetics at a professional level. Entering science in the 1960s, I could see the effect of that gap. One evening in 1968, a leading Russian biochemist was giving advice to an undergraduate: "Ah, Alex, don't waste your time on genetics. It's mostly obsolete. Beautiful in a strange way, but next to useless. They keep tormenting fruit flies, but it's us biochemists who will produce the understanding that really matters." Having spent a day reading genetic papers, I sensed he couldn't be right, that genetics was essential too. But that was just a hunch on my part, not the obvious truth that it would have been in a more enlightened setting.
Khrushchev, the successor to Stalin, continued to support Lysenko, but Khrushchev ran a "milder" dictatorship. Leading Soviet physicists, whose cachet with the government derived from physics' importance in military technology, helped Vladimir Engelhardt, a distinguished Russian biochemist, to organize a new research institute in 1959. Its initial name was the "Institute of Radiation and Physico‑Chemical Biology", since the term "molecular biology" was still verboten. The place was renamed as the "Institute of Molecular Biology" (IMB) in 1965.
At the Moscow University, both I and people around me knew that "real" molecular biology was being done largely in the West. Russian laboratories were strapped for everything – hard currency (rubles were worthless outside Russia), clean reagents, good equipment, and contacts with Western scientists. Promotions were based on merit once in a blue moon, while a capacity for intrigue and a membership in the Communist Party were particularly handy. I knew that my scientific milieu was far from first-rate but I was spared, for a while, the full comprehension of a gulf in quality between Eastern and Western molecular biology. One reason for a slow awakening was the sheer appetite for work: even washing dishes in the lab was not a chore to shirk from if it could accelerate an upcoming experiment.
After graduating from the university, I got a job at the Institute of Molecular Biology (IMB), a flagship place for doing such biology in Russia. The lab I worked in was led by Georgii Georgiev, one of the best scientists at the Institute. (Before having been hired, I moonlighted as an unpaid worker in the same lab for more than a year, while still an undergraduate at the university.) We studied chromosomes and RNA. Work was interesting, but it was difficult to ignore an undercurrent of scientific provinciality in my larger surroundings. What sustained me was youth, love of science, and ambition, a heady mix. It kept me working and hoping that things might improve. The time was the mid-1970s, a couple of years away from a chance to escape it all.
"Doing science is like driving a car at night. You can only see as far as your headlights, but you can make the whole trip that way." Long before I encountered this metaphor, by E. L. Doctorow (he wrote "Writing a novel", not "Doing science"), I sensed the attraction of the scientist's struggles: an air of open-ended adventure, a contest of sorts. The landscape is rough, changing, unpredictable. Other cars are racing toward Holy Grails out there, and occasionally collide with yours, by accident or not quite. A rambunctious life, but quiet on the outside, with maelstroms and lava flows hidden from casual view. A set of qualities for such a life must contain a genuine interest, beyond mere curiosity, in understanding the world's design, but an ambition as well, even with thinkers whose visage suggests otherwise.
Photos of old Einstein, a serene photogenic sage, free of strife, do not recall the assertive and ambitious young man in Switzerland, on the cusp of initial success, and his later, often overt, competition with rivals, a great German mathematician David Hilbert among them. Hilbert produced (and correctly interpreted) the equations of general relativity simultaneously with Einstein, a fact unmentioned in popular accounts of the subject but known to those who are interested in the history of physics. What Mad Pursuit is the title, from a poem by Keats, that Francis Crick chose for his autobiography. And mad it is, propelled not only by interest in understanding the world but also by desire to impress and awe – oneself, others, posterity.
ZIERLER: Alex, when did you first consider the possibility of leaving the Soviet Union, and how did you think such a feat would be possible?
VARSHAVSKY: By the 1970s, the idea of emigration was in the air, especially among Russian Jews, whose treatment by the regime was strikingly inconsistent. It was more difficult, though not impossible, for a Jew to become a student at a good college, more difficult to get a good job, and certain professions, particularly those linked to politics and power, were nearly closed to them. But, somehow, the very same Jews were allowed to apply for emigration to Israel and often actually left the country, a privilege denied to other ethnic groups in Russia, including Russians themselves.
The main causes of this policy by the government – discrimination and preferential treatment at the same time – are well understood: centuries of antisemitism in Russia, agreed with and abetted by authorities, but also their desire to curry favor with the West, in return for economic subsidies, direct or indirect.
The Jewish population of the Soviet Union, about three million in the early 1970s, did not pack up and leave altogether (though a lot of them did) owing largely to inertia, fears about life in another country, and also a significand likelihood of not obtaining the permission to emigrate. Such a refusal often meant the loss of one's job, harassment or worse.
The idea of attempting to leave the USSR occurred to me relatively late, a couple of years after graduation, in 1970, from the Moscow University. I loved my profession, was trying to succeed in it, and kind of forgot that a better way would be to change the place of work. My marriage history didn't help either. (It became even more checkered later on.) My first wife was my girlfriend in high school. I was 19 when we got married. Our daughter Victoria was born two years later, in 1968. By 1970, my wife and I were divorced. It would be flattery to call my first marriage a caricature of the real thing. Both of us were immature, and selfish in ways that children, not adults, tend to be. (These days, my daughter Victoria and her daughter Jenny, both of them accomplished professionals, live in London, U.K. My sister Marina and her large family live in the U.S., in Salt Lake City.)
I received a Ph.D. degree in 1973, and continued to work at IMB. Georgiev allowed me to supervise a graduate student and occasional undergraduates. My subject was the structure and dynamics of chromosomes, then largely a puzzle. This problem is huge, multifaceted, and remains a partially explored territory. In the early 1970s even "elementary" questions, about the path of DNA at the first level of its folding in chromosomes, and about the role that histones (abundant DNA-bound proteins) played in DNA folding, were unanswered. Our work during those years contributed, in a very minor way, to the problem's eventual solution, the discovery of nucleosomes, in 1974, by Roger Kornberg [17], an outstanding U.S. scientist who was working in England during the early 1970s.
My papers about chromosomes were published not only in Russian journals but in foreign ones as well. I began to receive invitations to give talks abroad, but attempts to receive permission to travel to Western countries were a waste of time. I was allowed, though, to visit a couple of other "socialist" countries, whose borders were sealed just as tightly as the borders of the USSR.
A stroke of luck, at first unrecognized, arrived in 1976. I received a letter from England, an invitation by Aaron Klug, an outstanding structural biologist in the U.K., to give a talk at a symposium in London that he was organizing. Despite knowing that permission to travel to London would be refused, I pretended, to myself, that things might not be totally hopeless, and requested an appointment with the Institute's director Vladimir Alexandrovich Engelhardt. He was in his mid-eighties then, a member of the Soviet Academy of Sciences and one of most highly positioned scientists in Russian biology.
Engelhardt, the founder and director of IMB, knew about me from the time of my joining Georgiev's lab in 1970, because my father headed another lab at the same institute. Georgiev spoke to Engelhardt about my work (or possibly work habits – I spent days and nights in the lab), and Engelhardt became interested. A relationship developed, utterly unequal but a real one. Engelhardt occasionally called me to his office and asked about ongoing work. I learned not to bore him with actual studies, for which his attention span was no longer equipped, and confined myself to just-so stories about chromosomes.
This time, I told Engelhardt about receiving the invitation from Klug to attend a symposium in London and to give a talk there. "I know Klug. Fine scientist, he. Hmm… I haven't been to England for some time. Are you the only one he invited from here?" I replied that I didn't know, but presumed that Klug invited only me. "Very well, then," said the old man. "Why don't we travel to London together, you and I?" "Vladimir Alexandrovich, I would be delighted to, but do you think this idea is realistic?" "Of course. Let's begin by sending a cable to Klug, and inquire whether he can send a formal invitation to me as well. Once we receive that invitation, I'll see what can be done. Please wait." I did, expecting refusal. A few months later, in February of 1977, I boarded the Soviet IL-62 for a flight, with Engelhardt, from Moscow to London.
I'll never know how he managed to obtain permission, from the directorate of KGB that handled such matters, for me to travel with him. My case was an open-and-shut one. A Jew, for starters. Divorced, i.e., "morally unstable": if he left his marriage, he can leave the motherland too. Known to the said directorate as someone who tells anti-Soviet jokes at Institute's parties. Besides, I didn't have hostages. In the Soviet Union back then, and in countries like North Korea today, a person trusted enough to travel abroad was expected to leave behind someone he or she would have difficulty parting with for good. Children, in a stable family, were the best hostages. A wife (or husband) was a so-so hostage but better than nothing. Parents were no hostages at all. By the logic of hostages alone, I shouldn't have been allowed to come anywhere close to that IL‑62. It must have been Engelhardt himself, his limited but tangible influence, that made the difference. Being the director of a major institute, he served officials above him, and kept the furnace of loyalty hot and burning for years. In return, he received occasional favors, such as taking his word that a nerd who didn't deserve to travel abroad and was a defection risk to boot, should be allowed to come with him.
Without telling anyone, I planned to defect in London, and was sure I would do it right after arrival. Then something happened to my resolve. The sight of old man who was kind to me and seemed to trust me; his fragility; and my concern that he may die from stress and disappointment if I defected were one reason, an attack of altruism if you will. There was also a smidgeon of fright, a reluctance to cut the knot irrevocably. In 1977, the Soviet Union appeared to me, and to everyone I knew, as a Thousand Year Reich. Leaving it would mean never meeting with my family and friends again, a price I thought was acceptable, then discovered it wasn't.
A week-long jaunt in England was over fast, a bewildering experience. I flew back to Moscow with Engelhardt, feeling miserable and believing, on good grounds, that I had lost my first and last chance of escape. The trip itself became a recollection. My talk at the symposium in London. Meeting scientists whose work I knew about. Traveling from London to Cambridge, where colleagues received me most kindly, and were generous with gifts of reagents and small gadgets for bench work. Walking in downtown London at night, marveling at shop windows and the profusion of lights in the streets. (Moscow was mostly dark at night.)
Having returned, I regaled acquaintances with tales of a life they never saw, but felt dejected, being sure I missed the only chance of escape. Then, a few weeks later, an utterly unexpected phone call: "Alexander Yakovlevich? My name is Vladimir Leonidovich. I'm a colonel at the Komitet Gosudarstvennoi Bezopasnosty." (Hence the acronym KGB.) "How was your trip to London? Good impressions? Lots of science, I gather." He continued before I managed to reply. "My colleague and I would like to meet with you next week, if you don't have objections." I certainly didn't. A few days later, I was going up in an elevator of the hotel Moskva (Moscow), near Red Square, having been instructed to knock at the door of a room on the seventh floor. Two men stood up to greet me, one of them in his forties ("Vladimir Leonidovich"; he never told me his last name), and a younger one, who introduced himself as "senior lieutenant". Both wore civilian clothes.
Our conversation, details of which I remembered for years but never wrote down, was lurching from one irrelevant subject to another. I played along, knowing they didn't invite me to hear interminable tales about catching huge pikes in the Oka river. They finally got tired of describing fishing exploits. Vladimir Leonidovich said, kind of jokingly, "I betcha you would like to travel abroad often, wouldn't you?" "Sure, who wouldn't," I replied, playing a level-headed fella, honest to a fault. "I enjoyed the visit to London, and my work at the Institute benefited from the trip." A proposition was soon advanced, simple and clear. "Look, Alexander Yakovlevich. We hear good things about you as a scientist. But not so good things about jokes at parties, ha‑ha‑ha!" He became serious and continued. "The Soviet Union and the entire socialist camp are surrounded by enemies. Counter-intelligence officers must know what the other side is up to. Genetic engineering – you know about that stuff, do you?" I nodded. "It may soon become an instrument in the hands of American military." To say "military", he used a quintessentially Soviet-Russian word, "voienschina": a military force to despise but also to fear. "We should be on lookout for these bastards' plans, to pre-empt them, and if necessary to develop countermeasures."
Countermeasures, my foot. From their remarks about molecular biology, in between guffaws and small talk, I knew that my counter-intelligence chums had a rather vague idea of what exactly DNA was, but they bravely pretended otherwise. Their orders from above were probably clear: to determine what exactly was that new imperialistic trick, genetic engineering. Not that anyone cared, with pikes, vodka and fishing gear looming larger in their minds than adenosine or thymidine. But reports had to be produced, "competent scientists" consulted, and gathered data recorded. The rusty machine creaked on, and invited me into its craw.
Everything went swell that day. I told them that my foremost duty as a Soviet citizen was to assist the counter-intelligence branch of "organs" (Russian slang for KGB) in its valiant efforts. To do the job, one must, naturally, travel to the West. That was fine, I was told, just fine, and we parted. This time, my good behavior on a trip would be vouched for by KGB officers I didn't care about, the mother of all understatements.
It so happened that before the visit to the Moskva hotel I received an invitation to give a talk at the international symposium on chromosomes in Helsinki, Finland. A few months rolled by. Near the end of August, 1977, I took the train from Moscow to Helsinki, Finland, a Western country. But not a good place for a political asylum, I was told by an acquaintance, in a casual conversation unrelated to my trip. The gist of his remark was that Finnish authorities were anxious to appease the USSR and therefore would return a (rare) defector, after giving him another chance to escape, this time from a Finnish police precinct. Whether or not that conjecture was actually correct I don't know to this day, but my taking it seriously made it necessary to cross, somehow, from Finland to Sweden. I didn't worry too much about it, though, because I counted on meeting a friend in Helsinki.
ZIERLER: Alex, what was the backstory and the considerations that made you consider the Finland trip as an escape route?
VARSHAVSKY: A year before my trip to Finland, Robert Hoffman, a young American scientist, came to IMB for a sojourn of several months, and began working there, sharing his time between IMB and a lab at the Moscow University. He was the first American I met for more than a few minutes. Gregarious, friendly, free, loving jokes, learning new language and taking delight in four-letter words that Russian is justly famous for, Bob Hoffman was a breath of fresh air.
It became obvious right away that Bob wasn't exactly a fan of the American political system. He described to me, a denizen of a deliriously happy country, the problem of unemployment in the U.S. and other such nightmares. Sensing that he is far more intelligent than his naiveté (about to be cured) suggested, I pulled no punches in telling Bob what I thought about the worker's paradise he decided to explore. Just two weeks of Bob's exposure to realities of the Soviet life produced a complete transformation. I was doing an experiment at the bench when Bob burst into the room and shouted "Alik!" ("Alex" is "Alik" in Russian.) "Do you know you're living in a fascist country?!" I said that I did, that now he knew it too, and if our neighbors on the floor didn't know it already they would have learned about it this very instant.
It didn't take long for Bob and me to become friends. We walked around IMB, discussing everything we could think of. I confided with Bob about the approach by the KGB, the impending trip to Helsinki, and my decision to escape from there to Sweden. Bob wholeheartedly approved of the plan, and suggested, with warmth and generosity of spirit – his particularly endearing trait – that he, too, would fly to Helsinki, but from Boston, to which he was about to return, being on leave from a lab at the Massachusetts General Hospital. Our idea was that Bob would travel to Helsinki and help me to escape.
We promised each other to keep mum about these plans. I knew the dates of symposium, and we decided, as characters in spy novels do, that Bob would wait for me at the Helsinki's railway station every hour on the hour during the symposium, for three days in the row. (The era of inexpensive long-distance phone calls was still years away.) I would try to find Bob at the railway station if I went to Finland, the permission being in the hands of my KGB handlers and still uncertain at that time.
Bob Hoffman and I met again in Helsinki, exactly as we planned. My talk was scheduled at the symposium for its first morning. In the afternoon of that day, there was a reception for participants. Just minutes after discussing nucleosomes and chromosomes with Francis Crick and his colleague Ruth Kavenoff, I went to the railway station. Bob Hoffman, having crossed the Atlantic to meet me, was there all right, sitting on a bench. He was conspicuous not only because of his height. The collar of his raincoat was straight up despite good weather, courtesy of spy thrillers.
Bob saw me, then looked around, checking for KGB agents with submachine guns. None of them showed up, having more interesting things to do. We smiled, laughed, and discussed the escape plan. A large ferry crossed the Baltic Sea between Helsinki and Stockholm every day. The idea was for Bob to buy two tickets to the ferry from Helsinki to Stockholm, departing the next morning, on the assumption that a ticket checker would be relaxed enough to let me in without a visa to Sweden.
The actual escape was nothing to write home about. A guard at the ferry, bored and indifferent, glanced at my ticket and waived me through. Once the ferry docked in Stockholm, we took a taxi and drove to the U.S. embassy. Its security officer was courteous but not exactly thrilled by our feat. He needed a Russian defector like a hole in the head. "I'll ship you to our Consulate in Frankfurt, Germany," he said. "It's a big place. There must be folks there who would assist you with getting a visa to the U.S."
Hours later, we were in Frankfurt. The U.S. Consul General there was too busy to receive me. A Consulate official, talking dishearteningly like bureaucrats I knew in the life left behind, explained that he could do nothing about the visa. Having escaped, I longed to begin scientific work as soon as possible. Therefore Bob and I decided that I would stay for a few days in Frankfurt, trying to get an audience with the Consul General.
Next day, we had another idea. Bob called David Baltimore at MIT in Cambridge, Massachusetts. The call stemmed from our shaky hope that Baltimore may have remembered me from my brief conversations with him at the 1975 USA-USSR symposium in Kiev, Ukraine, two years earlier. David was well known, already then, for his co-discovery of reverse transcriptase, a contribution for which he received the 1975 Nobel Prize in Physiology or Medicine. David remembered me, was friendly and gracious (in stark contrast to the official at the Consulate), and suggested that I should remain in Frankfurt for a few days, while he tried to find out whether anything constructive could be done about obtaining a visa.
In the meantime, Bob Hoffman had to go back to his job in Boston. He gave me money and left. I stayed at a seedy (as I realized only later) hotel in the Frankfurt's red-light district, a memorable experience for a runaway from a country with puritanical sexual mores. An audience with the Consul General didn't seem to be in the cards, but my mood couldn't be deflated by such a trifle. There was a Burger King nearby. I went there for breakfast, lunch and dinner (often followed by a second, late dinner), thinking that cheeseburgers and French fries belonged in the antechamber of Heaven, perhaps at the Heaven itself.
A couple of days passed. On the third day, a black limo made its way to the hotel along a narrow street. The Consulate's official emerged from it, and half an hour later I was ushered to the office of Consul General. He smiled at me and announced that he had in his hands a visa to the U.S. and an air ticket from Frankfurt to New York. First class, nonstop, all expenses paid. I flew first class again 15 years later.
The Consul General didn't mention the cause of such a startling reversal of fortune. I presumed (correctly) that our phone call to David Baltimore was involved. Long afterward, I learned that David called the office of Professor Frank Press, a distinguished MIT geophysicist who was, at that time, the science advisor to Jimmy Carter and had an office near the White House. My visa may have been cabled to Frankfurt from a place traceable to the White House. (I don't actually know.) This may account for the Consulate going overboard in sending me across the Atlantic immediately and in style (first class!), instead of in coach. Have arrived to New York, and from there to Boston. Bob Hoffman met me at the airport, and in no time at all I saw my first American apartment, Bob's, in Cambridge, near Harvard University. A nice place, with a sofa that became my bed.
Our first priority was to send a couple of cables to Moscow's IMB. The joy of having escaped was accompanied by concerns about possible repercussions for my parents and sister in Moscow, and for Georgiev and Engelhardt as well. My cables, written together with Bob, were designed to convey an image of a space cadet who didn't comprehend the hurtful seriousness of what he has done, and who even "hoped to return one day". It didn't matter whether or not the self-portrait, in those cables, of a bumbling knucklehead was believed by officials in charge of punishing people who remained behind. The incipient tiredness of the Soviet regime was reflected in its laid-back attitude toward transgressions that were not seen as a threat to state's security. The evidence, in the form of cables, that I was a loony would give officials a formal reason for relatively mild penalties, if they preferred to act that way.
I was denounced, in due course, at the IMB's public meeting. Engelhardt remained the director. Georgiev received a formal reprimand. My father was at first expelled from his membership in the Communist Party, but he was reinstated later. That reinstatement saved his job as an IMB professor. Everything went just about as well as it could, given what I've done.
ZIERLER: Where did you hope to gain a scientific appointment?
VARSHAVSKY: Right after my arrival in Cambridge, Massachusetts, in September of 1977, the laboratory of Bob Hoffman at the Massachusetts General Hospital, across the Charles river in Boston, became my second home. Bob and the lab's head Richard Erbe gave me a bench, and my work in the U.S. began. I was amazed by the luxury of having various supplies, things like disposable glass capillaries (precursors of tips in modern pipettes), clean napkins, helpful little gadgets, chemical reagents that were neither dirty nor difficult to obtain. In Moscow, I would have washed those capillaries with loving care, reusing them until the end of time.
A day or two later Bob and I visited the nearby MIT. We wanted to thank David Baltimore for his help with a visa to the U.S. I also hoped to meet with Alex Rich, another outstanding MIT scientist who traveled to Russia in earlier years and had visited IMB. David wasn't at MIT on that day, but Alex Rich was. He made a suggestion I didn't expect. "You traveled to Helsinki to attend a symposium," he said. "Ergo, you must have slides of your talk. Why don't you give us a seminar about your work in Moscow?" A week later I gave that seminar and was told it went well. The results I described, while not particularly exciting, were new and spanned a broad range, from nucleosomes to the folding of SV40 viral minichromosomes. Unbeknownst to me, MIT's Biology Department initiated a search for a junior faculty member in the arena of chromosome structure. Although my field of work was appropriate for the planned appointment, the idea of offering a faculty position to me must have looked odd, for I had fallen into Cambridge from the Moon, utterly outside of a formal search.
Just days after my MIT seminar, I received a call from Cyrus Levinthal, of the Columbia University in New York. He asked how I was and suggested to discuss the possibility of an assistant professor position at Columbia. I don't know what prompted Levinthal's call. Miracles kept piling up. Gene Brown, the genial chairman of MIT's Biology Department, told me that Francis Crick, whom I met for less than an hour in Helsinki (he also attended my talk there), has called Brown and inquired whether he could be of help in finding a job for me in the U.S. or U.K. A few days after that, Gene Brown offered me an assistant professor's position at MIT, less than two months after my leaving Moscow. I said "I accept" before Gene finished describing the offer.
What were the reasons for the lightning-fast transition from being a Russian runaway to a faculty member at MIT? The expectations of my U.S. colleagues were, most likely, far from high, given where I came from. It was, therefore, easier for them to be impressed by listening to a person who spoke a passably good English, presented new findings in the field of their interest, seemed to be in command of his craft, and also acted and sounded as someone utterly immersed in and excited by the already done work and studies ahead. They presumed, correctly, that it was much more difficult to do molecular biology in Moscow than in Cambridge, Massachusetts. It also didn't hurt that just a couple of months before the escape a detailed research paper about nucleosomes, by me and coworkers, was published in Cell [18], a leading journal in our profession.
A large room with seven lab benches and a small adjoining office was cleaned and ready for me at MIT in no time at all. I began working in my own lab, totally alone and happy as punch. When I needed research instruments, nearby labs allowed me to use theirs. I knew how to use a majority of the instruments, as some of them at IMB in Moscow were imported from the West. Gene Brown signed my requisitions for consumable supplies. He also told me that I would be able to buy equipment and hire coworkers in a year or so, once I receive my first grant, which I was supposed to write and submit to NIH as soon as possible. When I joined the Department, I had no idea about "start-up" funds for equipment and personnel that a newly hired faculty member was supposed to receive as a matter of course. Not knowing about start-up funds, I did not request them and received none. Learning about that omission several months later, I did not feel less grateful to Brown and colleagues, for I knew that hiring me must have been a gamble for them.
Soon after I began working at MIT, a man from the CIA came for a visit, to debrief me about the path from Moscow to Cambridge, Massachusetts. When he heard that the room in which I met with KGB officers was at the Moskva hotel, he nodded his jaded head and said "Yah, Yah. Seventh floor, a room with an oil painting of a woman on the wall near window, right?" "Absolutely right," I replied, amused and impressed by the CIA's colossal erudition.
Talking with the CIA visitor was fun, in part because he knew bits and pieces of Russian and we tried to use it during conversation. But no one from the FBI came to talk with me. Later, however, I was told that an FBI man went around MIT's Biology Department, asking faculty members whether they thought I might be a Russian agent. One professor said to the inquiring gentleman (in retelling; I didn't hear it myself): "Look, if Varshavsky is a spy, he must be an outstanding one. Why? Because he doesn't look like a spy. He looks like a schlemiel, which he might be. Leave him alone." ("Schlemiel", in Yiddish, is an inept, clumsy person.)
My colleagues at MIT, particularly David Baltimore, Paul Schimmel, Malcolm Gefter, the late Alex Rich and the late Howard Green, were the source of help and support from the beginning. Roger Kornberg, the discoverer of nucleosomes, has been working, then, at the Harvard Medical School in Boston (I met him during the 1977 trip to London). Roger contacted me soon after my arrival in Cambridge and was of tremendous, warm-hearted support. Robert Horvitz, a geneticist who studied nematode biology, began his work as an assistant professor at MIT almost simultaneously with me. His science went from strength to strength and was a great example.
In the excitement of my initial months at MIT, I managed to forget that Bob Hoffman may need some privacy, as he eventually told me, most tactfully, seeing that I didn't grasp, as yet, the advantage of living in my own apartment, rather than his. He also explained the upsides of sleeping on a real sheet, with a real blanket, as distinguished from rags on Bob's sofa. Having heard this, I encountered yet another miracle in my new country, for it took me an hour to rent a place in Cambridge. My apartment was a walk-up one-bedroom joint, in a creaky two-story house that was so infested with cockroaches (as I discovered later) that getting rid of them was a waste of time, as fallen cockroaches were swiftly replaced by cousins from adjoining flats. I was taken aback, a little, by those insects, as they were absent in my parents' Moscow apartment. But nuisances of everyday life barely registered. Work was all I cared about, with the intensity even greater than in Moscow, where I managed to get married, divorced, went to parties and found other ways to waste my time. None of that in Cambridge, Massachusetts. The monk was determined and more single-minded than Savonarola ever was.
Having convinced myself that I was in a breathtakingly free country, where one can finally say what one thinks, I carried the license far and wide. Soon after beginning the work at MIT, I told Gene Brown that I did not wish to teach students. Gene listened to my pronouncement, muttered something under his breath, then collected himself and told me that things were simple: if I refuse to teach, I would be fired. That information put a stop to refusals, but it took some time before the notion of a "totally free country" got adjusted to reality's level.
The dislike of teaching was not about teaching itself, for I understood its importance. I begrudged the time. No science could be done while delivering a lecture, and no learning either, with my exiting the classroom having the same knowledge I possessed before entering. Worse than that, I grew excited while actually teaching. So the dervish was tired after a lecture, and had to catch his breath before returning to work, yet another delay.
My first year at MIT, when I worked alone, was happiness itself. I usually walked from my apartment to MIT in late morning, having returned home the night before as late as I could. I often sang aloud on the way to the lab, beginning another day of work I loved, with no bosses in sight and no shortages of equipment or reagents. I knew the experiments to do, or thought I knew, and some experiments kept succeeding, making me happy in ways I didn't know existed.
Being short of time, I ate in a hurry, mostly at self-service MIT joints or at McDonalds and Burger Kings nearby. Fast food was tasty enough. That regimen went on for a year, until the end of 1978, when I fell ill, most likely from avitaminosis and other nutritional disbalances. My regimen of cheeseburgers and French fries ignored fruits and vegetables. Save for a bout of infectious mononucleosis at 16, I was never seriously ill before, and was baffled by rapidly worsening health: aches in the joints, wracking cough, difficulty sleeping, and frequent colds. I was rescued by a kind woman named Elena Erez, also an emigrant from Russia. She saw the cause of my condition better than I, guided me to healthier food, and cooked for both of us. At the beginning of 1979, she moved to my Cambridge apartment.
I soon recovered and resumed the hard work at MIT. Elena and I were discovering, gradually, that ours wasn't a durable union when she started to feel unwell, near the end of 1979. After several months of uncertainty, an aggressive cancer, leiomyosarcoma, was found. At the time of diagnosis we were unmarried, but got married soon afterwards. Elena was educated (though not medically) and intelligent, but a diagnosis of cancer often diminishes one's rationality, especially if remedies are meager or nonexistent. I tried to hide the truth from Elena, describing spontaneous remissions and other optimistic stuff. But knowing or at least suspecting that hers was metastatic cancer with a poor prognosis, she wanted to try her luck with "healers" in the Philippines, who claimed to cure cancer and most other diseases through "operations" done with bare hands, without knives. Booklets that Elena received described that and more. The healers were priests or claimed to be such, and had their base of operations in Baguio, a city not far from Manila, the capital of Philippines.
We flew to Manila in early 1982, drove to Baguio, and stayed at a hotel owned by the racket that the "priests" ran. I expected charlatanism, but was still unprepared for its brazenness, and for the willingness of patients to be duped by "operations", a bunch of low-grade tricks that shouldn't have deceived anyone. The patients (many of them from Europe and the U.S.) were ill adults, like Elena, or parents with sick children, paying for every "operation", of which there were always "many". Unable to offer Elena a cure from her illness, I felt obliged to keep my view of the place to myself. Elena enjoyed living at a tropical resort and seemed not to notice that the healers were mountebanks. After two weeks in Baguio, I had to go back to MIT, while Elena stayed at the hotel, where she preferred to be, saying that "treatments" were helping her. Three months later, I flew to the Philippines again, and brought Elena home. She knew that her condition was getting worse. My mantra about spontaneous remissions, while politely listened to, was probably no longer believed. A course of utterly ineffective chemotherapy at Boston's Beth Israel Hospital ensued. It was recommended to Elena by oncologists who (I bet) knew as well as I did that their toxic brew wasn't any better (actually worse) than useless but harmless "operations" in Philippines. Elena died in October of 1982.
I kept working at MIT throughout that time, to the extent I could. By about 1980, I had a functioning laboratory.
My later private life did not become serene, as I kept entering into marriages. The third one in 1985, the fourth in 1990. That (last) marriage ended in 2017. I understand, kind of halfway, the reasons for the later marriages, but a discussion in this domain would take us so far we may never return.
What follows is a semi-technical discussion of my lab's scientific work. The last section of the interview is non-technical.
ZIERLER: Alex, let's engage in a more technical discussion. What were your main scientific interests by the time you had you lab at MIT up and running?
VARSHAVSKY: By the beginning of 1978, I learned how to apply for grants. It wasn't difficult, just cumbersome and time-consuming. As described earlier in this interview, the competition for NIH grants, while considerable, wasn't as cutthroat as it is today. By 1982, the lab was funded by two NIH grants.
Discovery of exposed (nucleosome-depleted) regions in chromosomes
In Moscow, at IMB, I studied chromosome structure. During the first year at MIT, I worked alone and focused on the Simian Virus 40 (SV40) as a model of cellular chromosomes. The circular ~5,000 bp (~5 kb) minichromosome of this DNA virus can be isolated from infected green monkey (CV1) cells in culture. I tried to address the problem of nucleosome distribution on SV40 DNA in vivo. Nucleosomes, the repeating "subunits" of chromosomes, comprise two superhelical turns of DNA wrapped around the octamer of histones H2A, H2B, H3 and H4. Nucleosomes were discovered in 1974 by Roger Kornberg [17]. I wanted to determine whether the nucleosomes were distributed in a pattern that was nonrandom vis-á-vis specific nucleotide sequences of DNA. Or was their arrangement quasi-random, perhaps, in addition to being dynamic? To reduce potential nucleosome "sliding" on DNA (nothing was known about that aspect of nucleosomes at the time), I crosslinked isolated SV40 minichromosomes with formaldehyde (HCHO), a reagent that formed both protein-protein and protein-DNA crosslinks. My next step was to treat HCHO-crosslinked minichromosomes with restriction endonucleases that would be able to cut the naked (protein-free) SV40 DNA either just once or at several specific sites. Until these experiments, restriction endonucleases were not used with DNA‑protein complexes such as isolated chromosomes.
At first, I learned little. A bit later I saw that one DNA segment in the minichromosome was particularly susceptible to the cleavage by a single-cut endonuclease such as EcoRI. Remarkably, this "hypersensitive" region resided in a regulatory segment that contained the origin of SV40 DNA replication and viral transcriptional promoters. Soon thereafter, I did an analogous test with the multi‑cutter endonuclease HaeIII and hit a jackpot: the entire ~400-bp regulatory segment of the minichromosome could be "excised", by HaeIII, from an HCHO-crosslinked minichromosome as a fragment of protein-free DNA, in contrast to the rest of the ~5 kb minichromosome, which continued to be an intramolecular aggregate (despite multiple HaeIII cuts in its DNA), held together by HCHO-produced DNA-histone and histone-histone crosslinks.
These and related experiments produced two main insights: that the ~400 bp regulatory region of the SV40 minichromosome was strikingly more "exposed" (i.e., more susceptible to cuts by a sequence-specific endonuclease) than the rest of the ~5 kb minichromosome. In addition, the nucleosomes (including histones themselves) were apparently absent in the exposed ~400 bp region, in contrast to the rest of the minichromosome. More accurately, histones were either absent from the exposed region or were in a configuration that precluded histone-DNA crosslinks [19, 20].
Our first results were published in a 1978 paper [19]. A more detailed account was published in 1979 [20]. Two other groups, Carl Wu and Sarah Elgin at Harvard, and Walter Scott at the University of Florida, independently detected nuclease-hypersensitive regions in chromosomes, using a different approach that involved the accessibility of DNA in chromosomes to relatively nonspecific deoxyribonucleases such as DNase I. These simultaneous discoveries, by three labs, became a part of the modern understanding of chromosome organization. Later studies by many groups, including our lab, have shown that nuclease-hypersensitive (nucleosome-depleted) chromosomal regions, which facilitate an access to DNA in the otherwise tightly coiled chromosomal fibers, are a universal feature of chromosomes (including viral minichromosomes) at the origins of DNA replication, at transcriptional promoters, and at other regulatory sites.
Discovery of the DNA multicatenane pathway of chromosome cohesion/segregation
Near the end of 1978, I was reading a paper on minichromosomes and noticed a faint "ladder" of bands in a pattern of electrophoretically fractionated SV40 DNA. The paper's authors did not comment on those (barely visible) bands. At that time, Olof Sundin, a graduate student who recently joined my lab, began to study replication of SV40 minichromosomes and saw what appeared to be a similar set of minor DNA bands. (SV40 DNA replication intermediates can be detected by pulse‑labeling them, in vivo, with 3H-thymidine.) Neither of us suspected, then, that the study we were about to begin would take nearly three years and would lead, in 1980-1981, to the discovery of the first pathway of chromosome cohesion/segregation (Fig. 1) [21, 22].
When a circular minichromosome such as the one of SV40 virus begins its replication, two replication forks form, and run from the origin of replication in opposite directions, meeting halfway around the circle and leaving behind two circular daughter minichromosomes. Analogous processes take place during replication of cellular chromosomes, which are much larger, linear, and contain multiple origins of replication, so that a moving replication fork eventually meets a fork running toward it from an adjacent origin of replication. This level of understanding of chromosome replication faced a difficulty that wasn't even recognized as a problem at that time (the late 1970s): how do the two converging replication forks (large nucleoprotein structures containing DNA and RNA polymerases, helicases and many other proteins) replicate the last several hundreds of DNA nucleotide pairs that the forks themselves occupy?
Sundin and I discovered that before the replicated SV40 daughter minichromosomes separate from each other to yield individual minichromosomal circles, they go through a set of remarkable molecular transitions (Fig. 1). First, there is an increase of catenation (intertwining) of the two replicated DNA circles. Second, there is also a change, though quasi-independent enzymatic reactions, of the state of individual replication-produced DNA circles. They are initially nicked (i.e., contain a single-stranded cut or cuts in DNA) but become covalently "continuous" (covalently closed) circles as specific cut-sealing reactions take place in vivo (Fig. 1). The entire cohesion/segregation pathway begins with multicatenated minichromosomes, in which two ~5 kb replicated circular chromatids (daughter DNA circles) are intertwined around each more than 30 times! (It was the set of replicated and multicatenated pairs of SV40 DNA molecules which formed those faint electrophoretic "ladders" of bands that initially attracted my attention in 1978, in the absence of understanding of what those bands signified.)
The multicatenation stems, at least in part, from the in vivo conversion, by advancing replication forks, of the Watson-Crick intertwinings between two single strands of the parental DNA double helix into the topologically equivalent (but geometrically distinct) intertwinings of two double helices of the daughter DNA circles. This discovery revealed the first pathway of chromosome cohesion/segregation (Fig. 1) [21, 22]. Multicatenanes were a new form of double-stranded DNA at the time, inasmuch as only singly intertwined DNA circles were detected before 1980. It is possible that the multicatenanes we observed were formed not only at the termination of minichromosome replication but during replication as well, an important issue that our work did not address.
As described in the legend to Fig. 1, the minichromosome segregation in vivo is not a "linear" sequence of steps. Instead, it is a matrix of transitions that involves not only gradual decatenations but also a concurrent, quasi-independent sealing of gapped or nicked DNA duplexes in sister SV40 minichromosomes, so that the final (segregated) products of DNA replication are separate circles of the covalently closed SV40 DNA (Fig. 1). In vivo, the bulk of DNA is wrapped around histone octamers, forming nucleosomes. Because of this and other structural confinements (conformational constraints) on the folding of DNA double helices, those circular minichromosomes (i.e., complexes of DNA with histones and other proteins) in which DNA is nicked or gapped are conformationally similar, in vivo, to their covalently closed counterparts, with superhelical turns of DNA as a part of (constrained) nucleosomal arrays. In contrast, purified, histone-free DNA circles are relaxed if they contain a nick (or a single‑stranded gap) but are negatively supercoiled if they are covalently closed, since the latter form of protein-free DNA cannot dissipate its torsional strain through the rotation of DNA double helix (Fig. 1) [21, 22].
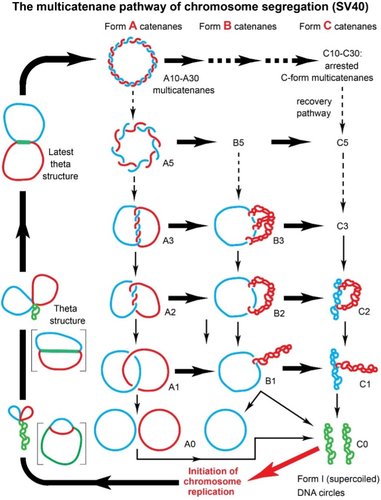
At the time of our work, type-II DNA topoisomerases, enzymes that mediate DNA decatenation (Fig. 1) and thereby make possible the segregation of chromosomes, were a novelty, having been identified, in the form of DNA gyrase, by the laboratory of Martin Gellert in the late 1970s and characterized by his and other laboratories, notably those of James Wang, Bruce Alberts, and the late Nicholas Cozzarelli. One aspect of our findings with SV40 minichromosomes was that type‑II topoisomerases became recognized as being also essential for decatenation of multiply intertwined chromosomal fibers (sister chromatids) that formed during chromosome replication [21, 22]. Genetic evidence that topo-II was indeed required for separating intertwined chromosomes via the multicatenane cohesion/segregation pathway (Fig. 1) were produced by several labs around 1985 [23-25]. In 1984, Steck and Drlica isolated a temperature-sensitive (ts) mutant of DNA gyrase in E. coli, and demonstrated a double-nucleoid chromosome segregation arrest of this mutant at nonpermissive temperature [26]. These and other findings showed that the multicatenane cohesion/segregation pathway (Fig. 1) [21, 22] was present and functionally essential in both eukaryotes and prokaryotes.
In our second "multicatenation" paper [22], Sundin and I proposed that the multicatenane pathway acts on multiply intertwined sister chromatids in ways that are likely to be controlled spatially, i.e., separately at specific chromosomal regions such as, for example, the centromere versus the arms of a chromosome, and temporally as well, i.e., at specific stages of mitosis or meiosis. Later work by others ([27-31] and references therein), including the evidence for a decatenation checkpoint, supported our early conjectures [22], and also revealed functional interactions between the multicatenane cohesion/segregation pathway (Fig. 1) and other processes, including checkpoints that monitor the completion of specific steps in the cell cycle.
The multicatenane pathway (Fig. 1) formally suffices to account for the sister chromatids staying together until their catenations have been resolved, stepwise, by type-II topoisomerases. However, there is also a separate, mechanistically distinct cohesion/segregation system, mediated by remarkable proteins termed cohesins. The cohesin-based pathway was discovered and analyzed during the 1990s by the labs of Douglas Koshland, Kim Nasmyth, Mitsuhiro Yanagida and Tatsuyo Hirano, with contributions by other groups as well. These findings included the identification of multisubunit cohesin proteins, which form circles around replicated daughter chromosomes [32-36]. This brief description of the cohesin system doesn't do justice to its importance, complicated designs and regulation. There is a functional and mechanistic complementarity between the multicatenane-mediated (Fig. 1) and cohesin-mediated cohesion/segregation pathways. Both pathways provides mechanisms for the adhesion and release of sister chromatids, and both pathways are essential for cell viability [37-40].
Chromosome segregation and protein degradation
During the early 1980s, my laboratory was becoming swamped by expanding ubiquitin (Ub) studies, which we began in 1978. Therefore, to my regret, I could not continue DNA multicatenane studies after 1982, and did not expect to return to the cohesion/segregation field. However, nearly two decades later, in 1999, Hai Rao (then a postdoc in the lab) and I saw, in a paper by Uhlmann, Nasmyth and colleagues [41], that the C-terminal (Ct) fragment of a separase-cleaved cohesin subunit contained the N-terminal (Nt) arginine (Arg) residue, which our lab's previous work had shown to act as a degradation signal (degron) in short-lived proteins. Such proteins were recognized by the Ub-dependent Arg/N-degron pathway (prior to 2019, this proteolytic system was called the "N-end rule pathway") (Figs. 3G and 4) [42, 43]. Rao and I decided to determine whether the separase-produced Ct-fragment of cohesin was, in fact, short‑lived in vivo, and if so whether its degradation was mediated by the Arg/N-degron pathway. By 2001, our collaboration with Uhlmann and Nasmyth demonstrated that the Arg/N‑degron pathway was indeed the one that targeted for degradation the separase-generated Ct-fragment of a cohesin subunit [44]. This degradation was also shown to be essential for high‑fidelity chromosome segregation [44]. Other colleagues, particularly Douglas Koshland, have previously discovered that "upstream" events, including the activation of a protease called separase (it cleaves the above cohesin subunit), are also regulated by the Ub system.
Discoveries that gave rise to the ubiquitin field
Given the number of specific and complementary discoveries, during the 1980s, by the laboratory of Avram Hershko (Technion, Haifa, Israel) and by my laboratory, then at MIT, that co-founded the modern Ub field, this part consists of three sections. The first one, a highly condensed summary of specific contributions, is directly below. It is followed by a brief introduction to the contemporary understanding of the Ub system, with an emphasis on N-degron pathways. The third section is a more detailed account of our initial and later Ub studies. For historical reviews of the early Ub field, see [45-48].
Fig. 2 and its legend describe the overall design of the Ub-proteasome system (UPS).
Fig. 3 and its legend describe the known N-degron pathways of protein degradation. They were discovered between 1986 and 2018 ([43] and references therein). In eukaryotes (but not in bacteria), these proteolytic systems are a part of the Ub system. Prior to 2019, N-degron pathways were called "N-end rule pathways" [43].
Fig. 4 and its legend describe the mammalian (e.g., human or mouse) Arg/N-degron pathway ([43] and references therein). A (simplified) diagram of the yeast (Saccharomyces cerevisiae) Arg/N-degron pathway is in Fig. 3G.
Fig. 5 and its legend describe the regulation of peptide import in S. cerevisiae by the Arg/N‑degron pathway [49-53].
- Discovery of ubiquitin-dependent protein degradation in vitro (in cell-free settings) by the Hershko laboratory (1978-1983)
The previously known ATP-dependent degradation of proteins in extracts from rabbit reticulocytes was discovered, by the laboratory of Avram Hershko, to involve a new protein modification, the covalent conjugation of ubiquitin (Ub), a 76-residue protein, to other proteins in these extracts, including test proteins added to an extract [45, 54, 55]. Ub-protein conjugation (ubiquitylation) was shown to be mediated by specific enzymes, which were isolated and termed, by Hershko and colleagues, as E1, E2 and E3. These insights were produced using in vitro (cell‑free) systems and isolated E1-E3 enzymes [45].
The E1 (Ub-activating) enzyme was shown, by Hershko and colleagues, to generate an activated form of Ub, bound to a specific Cys residue of E1 via a thioester bond. They also showed that the E1-linked activated Ub moiety is transferred, via transesterification, to a specific Cys residue in one of several E2 (Ub-conjugating) enzymes. Their further analyses showed that an E2 enzyme, in a functional cooperation (mechanistically undefined, at the time) with one or more of E3 proteins, transferred the activated Ub moiety from an E2 enzyme to the ε-amino group a specific Lys residue in an "ultimate" substrate protein [45]. The identities and mechanisms of E3 proteins were understood significantly later (see below).
Substrate proteins that were ubiquitylated in vitro under the above conditions were degraded, in the extract, by an ATP-dependent protease that was characterized by other laboratories much later (starting in the early 1990s), and is now called the 26S proteasome [45-48, 56, 57].
2. Discovery of degradation signals and biological fundamentals of the ubiquitin system by our laboratory (1984-1990)
The first degradation signals (degrons), i.e., specific features of proteins that mediate their degradation in vivo, were discovered in 1986 by our laboratory [58, 59]. The term "degron" was proposed by us in 1991 [60]. Over the last decades, it became a standard term in the field. The first degrons to be discovered were N-terminal (Nt). They were called N-degrons [42, 43, 60-62]. The main determinant of an N-degron in a protein is its destabilizing Nt-residue.
The Ub-dependent proteolytic system which targets specific N-degrons was called, in 1986, the N-end rule pathway [58, 59]. Much later, in 2019, this term was replaced, with the concurrence by other groups in the field, by the term the "Arg/N-degron pathway", inasmuch as other (analogous but distinct) N-degron-based proteolytic systems were also discovered, by us and by other groups, between 2010 and 2018 (Figs. 3 and 4) (reviewed in [43]). Until 1986, the nature of degradation signals (degrons) in short-lived proteins was unknown. Discovery of the first degrons [58] solved the fundamental problem of selectivity in the Ub system.
In other (parallel and independent) studies by our lab during 1984-1990, my colleagues and I discovered that ubiquitylation of proteins has strikingly broad in vivo functions, largely through the control, via processive proteolysis, of the levels of many intracellular proteins, and also through pathways that involve Ub but do not necessarily involve proteolysis.
Specifically, we have shown that ubiquitylation is essential for the bulk of protein degradation in living cells (1984) [63, 64]. We also discovered the first specific functions of ubiquitylation: in DNA repair (1987) [65]; in the cell cycle (1988) [66]; in stress responses (1987) [67]; in protein synthesis (1989) [68]; and in transcriptional regulation (1990) [69].
We also identified the first physiological substrate of the Ub system (the MATalpha2 transcriptional repressor) [69]. The first genes encoding precursors of Ub (linear poly-Ub and fusions of Ub to specific ribosomal proteins) were identified and cloned by us in 1984-1989 [68, 70, 71]. The first Ub-conjugating (E2) enzymes with specific biological functions, RAD6 (in DNA repair) and CDC34 (in the cell cycle), were identified by our lab in 1987-1988 [65, 66]. In 1991-1992, my colleagues and I cloned the first deubiquitylating enzymes, termed UBP1-UBP3 [72, 73].
In 1990, Bonnie Bartel and Ingrid Wünning (then, respectively, a graduate student and postdoc in our lab) identified and cloned the first specific E3 Ub ligase, termed UBR1 [74]. The molecular cloning of the first E3 Ub ligase opened up a particularly vast field, as later studies showed that the human genome encodes ~800 distinct E3 Ub ligases [74-76]. This enormous diversity of E3s, many of which recognize distinct degrons (see above), underlies the immense functional reach of the Ub system.
In 1989, Daniel Finley, Bonnie Bartel and I discovered the first nonproteolytic function of Ub, specifically its activity as a cotranslational chaperone in the biogenesis of ribosomes [68]. Also in 1989, Vincent Chau and colleagues in our lab discovered the first substrate-linked poly-Ub chains, with the isopeptide bonds between Gly-76 and Lys-48 of adjacent Ub moieties, as well as the necessity of substrate-linked (branched, as distinguished from linear) poly-Ub chains for the proteasome-mediated protein degradation [77].
In 1990, Erica Johnson and David Gonda (then, respectively, a graduate student and postdoc in our lab) discovered that the Ub system exhibits subunit selectivity, i.e., the ability to destroy a specific subunit of an oligomeric protein while leaving the rest of the protein intact. That, previously unknown, capability of the Ub system makes possible protein remodeling [78]. This capability underlies key aspects of the cell cycle (e.g., the replacements of cyclin subunits in cell‑cycle kinases), the activation of transcription factors such as, e.g., NF-kappaB, and a vast multitude of other processes.
Together, two sets of complementary discoveries, during the 1980s, that are described in item 1 (insights by the Hershko laboratory) and in item 2 (insights by our laboratory), have co-founded the modern Ub field and led to the enormous expansion of Ub research over the next three decades, through studies by many laboratories that entered this arena in the 1990s and afterward. Investigations of the Ub system, both fundamental and applied (Ub-related pharmacology), are now one of the largest fields in biomedical science, the point of convergence of many disparate disciplines.
Papers about the early history of the ubiquitin field
Hershko, A., Ciechanover, A. and Varshavsky, A. (2000) The ubiquitin system.
Nature Medicine 6, 1073-1081 [45].
Varshavsky, A. (2006) The early history of the ubiquitin field. Protein Science 15, 647-654 [46].
Varshavsky, A. (2008) Discovery of cellular regulation by protein degradation. J. Biol. Chem.
283, 34469-34489 [47].
Varshavsky, A. (2014) Discovery of the biology of the ubiquitin system. J. Am. Med.
Association (JAMA) 311, 1969-1970 [48].
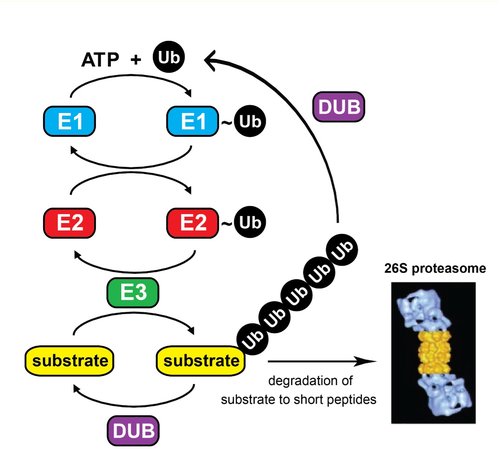
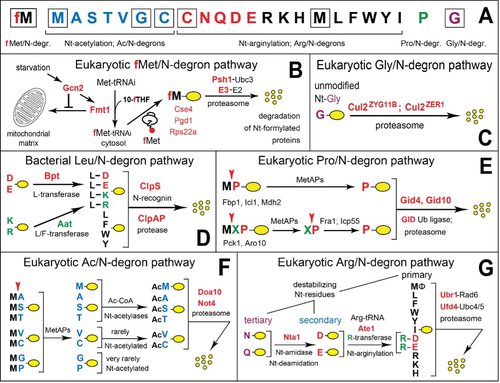
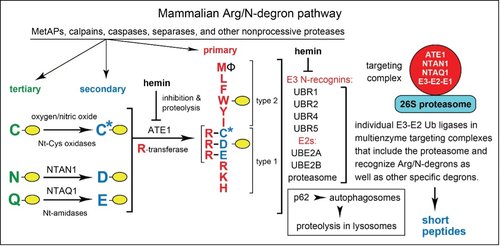
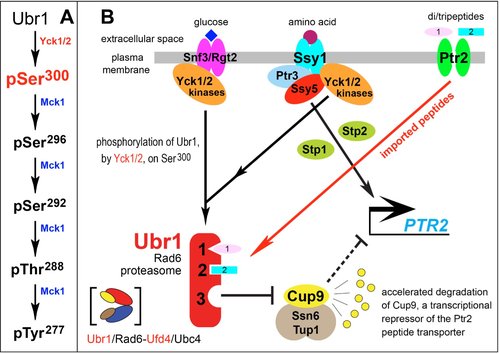
Introduction to the modern understanding of the ubiquitin system, with an emphasis on N‑degron pathways [43] and a mention of C-degron pathways
This brief and ahistorical introduction to the current state of the Ub field can be juxtaposed to the two historical accounts, above and below: the first section, above, is a condensed summary of contributions, during the 1980s, by the Hershko and my laboratories (items 1 and items 2 above). The third section, after the present one, is a more detailed historical account that includes later studies.
Regulated protein degradation protects cells from misfolded, aggregated or otherwise abnormal proteins, and also controls the levels of proteins that evolved to be short-lived in vivo. The intracellular protein degradation is mediated largely by the ubiquitin (Ub)-proteasome system (UPS) (Fig. 2) and by autophagy-endosome-lysosome pathways, with molecular chaperones being a part of both systems [43, 45, 88, 97, 154-159]. The UPS is a set of pathways that have in common two classes of enzymes: E3-E2 Ub ligases and deubiquitylases (Fig. 2). A Ub ligase recognizes a substrate protein through its degradation signal (degron) and conjugates Ub, a 9 kDa protein (usually a poly-Ub chain), to an amino acid residue (usually an internal lysine) of a targeted substrate. The functions of deubiquitylases include deubiquitylation of Ub-conjugated proteins [43, 76, 88, 97, 160, 161]. The 26S proteasome, an ATP-dependent protease, binds to a poly-Ub chain of a polyubiquitylated protein, unfolds it, and processively converts it to peptides that range from ~3 to ~25 residues (Fig. 2) [57, 162-166].
N-degron pathways (previously called "N-end rule pathways") are proteolytic systems that have in common their ability to recognize proteins containing N-terminal (Nt) degrons called N‑degrons, thereby causing degradation of these proteins by the 26S proteasome and/or autophagy in eukaryotes, and by the proteasome-like ClpAP protease in bacteria (Fig. 3) [42, 43, 58, 59, 100, 123, 128, 135-138, 146, 167-185]. Determinants of an N-degron include a destabilizing Nt-residue of a protein and its internal Lys residue(s) that functions as a polyubiquitylation site [43, 59, 186].
Eukaryotic N-degron pathways comprise the Arg/N-degron pathway (it recognizes, in particular, specific unacetylated Nt-residues); the Ac/N-degron pathway (it recognizes, in particular, the Nα‑terminally acetylated (Nt-acetylated) Nt-residues); the Pro/N-degron pathway (it recognizes, in particular, the Nt-Pro residue); the Gly/N-degron pathway (it recognizes the Nt-Gly residue); and the fMet/N-degron pathway (it recognizes Nt-formylated proteins) (Figs. 3 and 4) [42, 43, 142, 167, 168, 170, 171, 183-185, 187]. Bacteria also contain at least one N-degron pathway (it is Ub-independent), called the Leu/N-degron pathway (Fig. 3D) [43, 110, 114, 120, 188]
Initially, most N-degrons are cryptic (pro-N-degrons). They are converted to active N‑degrons either constitutively (e.g., cotranslationally) or via regulated steps. Many nonprocessive proteases, including Met-aminopeptidases, caspases and calpains, function as components of N‑degron pathways, since a cleavage of a protein can generate a destabilizing Nt‑residue in the resulting C‑terminal (Ct) fragment [128, 130, 183, 189]. Another route to active N‑degrons are enzymatic Nt‑modifications of proteins, including Nt-acetylation, Nt-deamidation, Nt-oxidation, Nt‑arginylation, Nt-leucylation, and Nt-formylation (Figs. 3 and 4) [43].
Recognition components of N-degron pathways, called N-recognins, are E3 Ub ligases or other proteins (e.g., bacterial ClpS or mammalian p62) that can target N-degrons [43, 146, 157, 167, 168]. All 20 amino acids of the genetic code can function, in cognate sequence contexts, as destabilizing Nt-residues [43]. Thus, many proteins in a cell are conditionally short-lived N‑degron substrates, either as full-length proteins or as protease-generated Ct-fragments.
Regulated degradation of proteins and their natural fragments by N-degron pathways has been shown to mediate a multitude of biological processes, including the sensing of oxygen, nitric oxide (NO), heme, and short peptides; the control of subunit stoichiometries in protein complexes; the elimination of misfolded proteins and of proteins retrotranslocated to the cytosol from other compartments; a suppression of neurodegeneration and regulation of apoptosis; the control of DNA repair, transcription, replication, and chromosome cohesion/segregation; the regulation of chaperones, G proteins, cytoskeletal proteins, autophagy, gluconeogenesis, peptide transport, meiosis, circadian rhythms, fat metabolism, cell migration, adaptive and innate immunity (including inflammation), the cardiovascular system, neurogenesis and spermatogenesis; and also plant defenses against pathogens, plant cell differentiation, sensing of oxygen and NO, and many other processes in plants ([42, 43, 58, 100, 120, 123, 129, 135-138, 146, 167-173, 175-184, 187, 190-192] and references therein).
In multicellular eukaryotes, including mammals, the Arg/N-degron pathway is mediated by at least four N-recognin E3 Ub ligases. Two of them, Ubr1 and Ubr2, are sequelogous (similar in sequence [193]) to each other and to Ubr1 of the yeast Saccharomyces cerevisiae (Fig. 4) [43, 74, 75, 142-144, 194, 195]. Ubr1, the sole N-recognin of the S. cerevisiae Arg/N-degron pathway, is a 225 kDa RING-type E3. In vivo, Ubr1 is a subunit of the double-E3 Ubr1-Rad6/Ufd4-Ubc4/5 complex. It contains, in particular, the Ubr1-bound 168 kDa Ufd4, the Ubr1-bound 20 kDa Rad6 Ub-conjugating (E2) enzyme, and either Ubc4 or Ubc5 E2 bound to Ufd4, a HECT-type E3. Ufd4 is not an N‑recognin [126]. Two other components of this multienzyme targeting complex are the Ate1 arginyltransferase (R-transferase), bound to Ubr1, and the Nta1 Nt‑amidase, bound to R‑transferase (Figs. 3G and 4) [142].
Unmodified N-terminal Arg, Lys, His, Leu, Phe, Tyr, Trp, Ile and Met (if Nt-Met is followed by a bulky hydrophobic residue) are "primary" destabilizing Nt-residues in that they are directly recognized (bound) by Ubr1 (Figs. 3G and 4) [42, 43, 58, 128, 130, 173, 175, 196-198]. In contrast, Nt-Asp and Nt-Glu are destabilizing owing to their Nt-arginylation by Ate1 R-transferase. The resulting Nt-Arg can be captured by Ubr1 Varshavsky, 2019 #4907. Nt-Asn and Nt‑Gln are destabilizing since the Nta1 Nt-amidase converts them to Nt-arginylatable Nt-Asp and Nt-Glu (Fig. 1) [108, 199].
The type-1 and type-2 binding sites of Ubr1 E3 bind, respectively, to basic (Arg, Lys, His) and bulky hydrophobic (Leu, Phe, Tyr, Trp, Ile) Nt-residues of proteins or short peptides. Ubr1 contains more than two substrate-binding sites, and therefore can target not only Arg/N‑degrons but also other degradation signals in yeast proteins that lack Arg/N-degrons. These proteins include Cup9, Mgt1, Chk1, Kar4, Tup1, Gpd1, Ste11, and Hsc82 [51, 52, 137, 192].
Ct-degradation signals, called C-degrons, are present in both eukaryotic and bacterial proteins, and are targeted by proteolytic systems called C-degron pathways [43, 200-203]. The main determinant of a C-degron is a destabilizing Ct-motif that often includes the protein's Ct-residue. A broad range of C-degrons that are targeted by Ub ligases of the cullin-RING (CRL) family has been identified in mammals [43, 200-203]. In addition to being topologically analogous, N‑degrons and C-degrons are related functionally. For example, a proteolytic cleavage of a subunit in a multisubunit complex can create, simultaneously, a new N-degron (in a subunit's Ct‑fragment) and a spatially adjacent new C-degron (in the sibling Nt-fragment). Consequently, both fragments of the cleaved subunit can be selectively destroyed (sparing the rest of the complex), through attacks by N-degron and C-degron pathways [43, 78, 200-203].
This summary of the field's current state can be juxtaposed with the section below, a historical account of studies by our laboratory, during the 1980s, that discovered degradation signals (degrons) and biological fundamentals of the Ub system.
Discovery, during the 1980s, of degrons and biological fundamentals of the ubiquitin system [45-48]
As described above, I began working at MIT in the fall of 1977, shortly after leaving the former Soviet Union. At that time, I studied chromosomes. I was also interested in proteases and proteolysis, in part because I saw that field as important but largely obscure in its most interesting part, the regulated degradation of proteins in vivo, in living cells. However, I was also interested in other biological problems, and did not suspect that studies of protein degradation would soon displace just about everything that my nascent lab was trying to do.
The libraries, which I loved in Russia, were just as quiet and pleasant in Cambridge as they were in Moscow. A library at MIT soon became my second home. Reading there, I came across a 1977 paper by Ira Godknopf and Harris Busch [204]. They found a DNA-associated protein that had one C-terminus but two N-termini, an unprecedented structure. The short arm of that Y‑shaped protein was joined, through its C-terminus, to a specific internal Lys residue of histone H2A, one of the four "core" histones, the protein components of nucleosomes. The short arm of the Y-shaped protein was soon found, by others, to be Ub, a 76-residue protein of unknown function that had been identified (as a free protein) in 1975 by Schlesinger and colleagues [205].
I became interested in that first Ub conjugate, Ub-H2A. In 1975, when I was still in Russia, we developed a technique for high-resolution fractionation of mono- and oligonuclesomes, produced by treatments of isolated chromatin with staphylococcal nuclease. This electrophoretic technique, a forerunner of gel-shift assays, was based on polyacrylamide gel electrophoresis of DNA‑protein complexes in a buffer of a low ionic strength [206, 207]. At MIT, Louis Levinger (then a postdoc) and I advanced this method further by adding an SDS-gel electrophoresis in the second dimension [208, 209]. A first-dimension gel separated mononucleosomes into three subspecies. In addition to a ~145 bp fragment of DNA and the histones H2B, H3 and H4, these subspecies contained, respectively, two molecules of histone H2A, one molecule each of H2A and Ub-H2A, or two molecules of Ub-H2A [210].
In our approach, DNA and protein components of the nucleosomes that were fractionated in the first dimension were dissociated within a gel slice and thereafter electrophoresed in the second dimension, using an SDS-containing gel. That step was followed by Southern hybridization with 32P-labeled DNA probes [208]. In 1982, we applied this new method, 2-D mapping of nucleosomes, to Drosophila chromatin, and discovered that a transcribed gene such as HSP70 was greatly enriched in the mononucleosomes that contained one or two molecules of Ub-H2A, whereas the centromeric heterochromatin (specifically its regions containing the 1.688 Drosophila satellite DNA) was completely devoid of ubiquitylated nucleosomes [209].
These 1982 Ub findings [209], two years prior to our genetic papers that initiated the biological understanding of the Ub system [58, 59, 63-71, 74, 77, 78, 106, 211, 212], were the first evidence bearing on biological functions of Ub itself and ubiquitylated histones. Decades later, in the current field of histone modifications, ubiquitylated histones H2A or H2B are a part of remarkable setting that encompasses not only ubiquitylation but also acetylation, methylation, sumoylation, ADP-ribosylation, citrullination and other modifications of specific histones. All or most of these changes are reversible in vivo. Histone modifications are "read" by specific chromatin-associated proteins of many kinds, including enzymes that modify histones as a function of their previous modification states and specific locations in chromosomes. Our 2-D mapping, 40 years ago, of ubiquitylated Drosophila nucleosomes, and the conclusion that ubiquitylation of histones plays a role in transcriptional regulation [209], are in agreement with current studies.
In the late 1970s, Avram Hershko, his graduate student Aaron Ciechanover and their colleagues in the Hershko laboratory at the Technion (Haifa, Israel) were studying ATP-dependent protein degradation in extracts from rabbit reticulocytes. In 1978, they discovered that a small protein, which they termed APF-1 (ATP-dependent proteolytic factor 1), was covalently conjugated to proteins before their degradation in the extract [54]. In 1980, they suggested that the APF‑1 moiety linked to another protein served as a signal for a downstream protease [55], and began to analyze the enzymology of APF-1 conjugation. In 1981-84, Hershko and coworkers identified a set of three enzymes involved, termed E1 (Ub-activating enzyme), E2 (Ub-conjugating enzyme) and E3 (an accessory component that appeared to confer specificity on E2) [213, 214]. A large ATP-dependent protease that mediates the processive degradation of Ub-protein conjugates [215] was characterized by several laboratories much later, in the early 1990s and afterward, and is now called the 26S proteasome (for recent reviews of the proteasome, see [56, 57]).
Although our studies of Ub (in chromosomes) began in 1978, I did not know about APF-1 results by Hershko and coworkers, because the identity of APF-1 and Ub was unknown, at that time, to them as well. This changed in 1980, when APF-1 and Ub were shown to be the same protein [216], by Keith Wilkinson, Michael Urban and Arthur Haas, who worked in the laboratory of Irwin Rose, a collaborator of Hershko during his stays at the Philadelphia's Fox Chase Cancer Center in the U.S.
When I read the 1980 paper of Hershko et al. that described the APF-1 conjugation to proteins in reticulocyte extracts [55] and the 1980 paper of Wilkinson et al. that described the identity of APF-1 and Ub [216], two previously independent realms – protein degradation and ubiquitylated nucleosomes – came together, suggesting a regulatory system of great complexity and broad but still to be discovered biological functions. I became very interested and decided to find genetic approaches to the problem, because a system of such complexity was unlikely to be understood through biochemistry alone. In 1980, reverse-genetic techniques were about to become feasible with S. cerevisiae, but were still ~15 years away in mammalian genetics.
Mouse ts85 cells and the first evidence about the biology of the ubiquitin system
I kept reading, as widely as I could. Near the end of 1980, I came across a short paper by the laboratory of M. Yamada in Japan that described a temperature-sensitive (ts), conditionally lethal mouse cell line called ts85. The researchers showed that a specific nuclear protein disappeared from ts85 cells at elevated temperatures [217], and suggested that this protein might be Ub-H2A. Glancing at their data, I had to calm down to continue reading, as I was nearly certain that the protein they saw was, in fact, Ub-H2A: in the preceding two years we had learned quite a bit about electrophoretic properties of this Ub conjugate. On the hunch that mouse ts85 cells might be a mutant in a component of the Ub system, I wrote to Yamada and received from him, in 1981, both ts85 cells and the parental ("wild-type") mouse cell lines.
Daniel Finley, then a graduate student, joined my lab at that time to study regulation of gene expression, but did not need much convincing to switch to a study of ts85 cells. A few months into the project, Finley and I made the critical observation that Ub conjugation in extracts from ts85 cells was temperature-sensitive, in contrast to extracts from parental cells. While this was going on, I met Ciechanover, who came from the Hershko laboratory in Israel for a postdoctoral stint at MIT and was working on unrelated subjects (growth factor receptors) in the laboratory of Harvey Lodish. Presuming that Ciechanover was still interested in Ub (very few people were, at that time), I told him about our results with ts85 cells, and invited him to join, part-time, with Finley and me to complete the ts85 study. Ciechanover joined us, the work continued, and in 1984 we published two papers that described two conceptually independent discoveries [63, 64]:
- Mouse ts85 cells have a temperature-sensitive (ts) Ub-activating (E1) enzyme;
- These cells, in contrast to parental cells, stop degrading the bulk of their short lived proteins at nonpermissive temperature.
These results were the first evidence that Ub conjugation was required for the bulk of protein degradation in vivo, in living cells. (The earlier studies by Hershko and coworkers were carried out with cell-free extracts from reticulocytes.) Our results [63, 64] also explained the disappearance of Ub-H2A from ts85 cells at nonpermissive temperature: thermal inactivation of the mutant (ts) Ub-activating (E1) enzyme in these cells stops de novo formation of Ub-H2A, while pre‑existing Ub-H2A is gradually deubiquitylated by constitutively active deubiquitylases. In a paper published in 1983 [218], Yamada and coworkers confirmed their initial conjecture about the identity of Ub-H2A as a protein that disappeared from ts85 cells, but pinpointed neither the cause of this effect (a ts E1 enzyme, as it turned out), nor, most importantly, the crucial property of these cells: their near-inability, at nonpermissive temperature, to degrade proteins that are normally short-lived.
Our results with ts85 cells [63, 64] also indicated that ubiquitylation was essential for cell viability, the first hint of the massive, wide-ranging biological importance of the Ub system. In addition, ts85 cells were preferentially arrested in the G2 phase of the cell cycle, and the synthesis of heat-stress proteins was strongly induced in these cells at the nonpermissive temperature (in contrast to parental cells), suggesting that Ub conjugation was involved in the cell cycle progression and stress responses [63, 64].
Two decades after our ts85 papers of 1984 [63, 64], the late Cecile Pickart, an early and important contributor to Ub research, had this to say about the novelty and impact of these studies: "The two papers ... led to a new world-view; not only was the ubiquitin pathway a major proteolytic mechanism in mammalian cells, but it was also likely to regulate the cell cycle. These conclusions are so well accepted today that it is difficult to appreciate the magnitude of their impact at the time the two papers appeared." (Pickart, C.M., Cell 116, 181(2004)).
Although our conclusions, from the results with mouse ts85 cells, about specific functions of the Ub system proved correct [43], they were still preliminary in 1984, given the limitations of mammalian somatic cell genetics. We decided, therefore, to turn to the yeast S. cerevisiae, where such issues could be addressed more rigorously. As described below, our 1987-88 discoveries with the yeast RAD6, CDC34 and UBI4 proteins [65-67] produced the first specific and definitive evidence that the Ub system is of central importance for DNA repair, the cell cycle, and stress responses. In 1983, Tim Hunt and colleagues identified unusual proteins in sea urchin and clam embryos [219]. These proteins, which were termed cyclins, were found to be degraded at the end of mitosis [219]. In our 1984 papers, we suggested that cyclins were conditionally destroyed by the Ub system [63, 64], a hypothesis shown to be correct in 1991, by Glotzer, Murray and Kirschner [220], and independently by Hershko and coworkers [221].
It may be helpful to place the above advances in historical context. Despite some evidence to the contrary, until the 1984 Cell papers about mouse ts85 cells [63, 64] and our later genetic discoveries with the Ub system of S. cerevisiae, the prevailing view was that intracellular protein degradation was largely about destruction (through unknown pathways) of damaged proteins. The bulk of cellular regulation was believed to be a separate affair, mediated primarily by repressors and activators of gene expression, which were assumed, often tacitly, to be long-lived. Among the reasons for this incorrect view were difficulties in connecting the long-recognized proteolytic system in the lysosomes to specific pathways of intracellular regulation.
Thus, most researchers studying gene expression in the 1960s and 1970s tacitly presumed that regulatory circuits they cared about did not involve short-lived proteins. As we now know, just the opposite proved true, particularly in eukaryotes, where most regulators of transcription are conditionally short-lived proteins whose levels in a cell are determined at least as much by the rates of their Ub-dependent destruction as by the rates of their synthesis through transcription and translation. Given the previously presumed stability of transcriptional regulators, it is ironic that the first physiological (as distinguished from artificial) substrate of the Ub system was MATalpha2, a transcriptional regulator that Mark Hochstrasser (then a postdoc) and I demonstrated in 1990 to be a short-lived protein in vivo [69].
In 1983, even before the ts85 studies were completed, Finley, Engin Özkaynak (then a postdoc) and I, together with other colleagues in the lab, began systematic analyses of the Ub system in the genetically tractable S. cerevisiae, a project that soon expanded to occupy the entire laboratory. Between 1983 and 1990, this work revealed the biological fundamentals of Ub conjugation and discovered the first degradation signals (degrons) in short lived proteins. These key advances of early years are described below.
A linear polyubiquitin gene and resistance to stress
In 1984, Özkaynak, Finley and I identified and cloned the first Ub gene in S. cerevisiae. Unexpectedly, it encoded not a single Ub moiety but a head-to-tail linear poly-Ub precursor protein [70]. By 1987, we showed that this gene, UBI4, was induced by a large variety of stresses, and that a deletion of UBI4 resulted in hypersensitivity to these stresses (stress-hypersensitive ubi4Δ cells were viable under normal conditions, as they contained other Ub genes as well) [67, 71]. These genetically based results validated and deepened our earlier indirect evidence with mouse ts85 cells [63], thus identifying stress responses as one important and broad function of the Ub system.
RAD6, DNA repair, and the ubiquitin system
In 1987, the late Stefan Jentsch (then a postdoc), John McGrath (then a graduate student) and I discovered that RAD6, a protein known to yeast geneticists as an essential component of DNA repair pathways, is a Ub-conjugating (E2) enzyme, the first Ub-conjugating enzyme with a specific physiological function [65]. In addition to learning that the Ub system is required for DNA repair, we noticed that the sequence of RAD6 was weakly similar to that of CDC34, an essential cell cycle regulator (of unknown biochemical activity) that had been identified genetically in S. cerevisiae by Lee Hartwell and had been shown to be required for the G1-S phase transition in the cell cycle. The 1987 RAD6 insight [65] opened up the field and led to studies by many laboratories that revealed, over the next decade, the massive, multilevel importance of the Ub system in DNA damage responses.
CDC34, the cell cycle, and the ubiquitin system
To verify the above possibility that CDC34 may also be a distinct Ub-conjugating (E2) enzyme, Jentsch, McGrath and I collaborated with Mark Goebl in the laboratory of Breck Byers (University of Washington, Seattle). In 1988, this collaboration demonstrated that CDC34 is indeed a specific Ub-conjugating (E2) enzyme [66], thereby producing the first rigorous proof that the Ub system is an essential part of the cell cycle. (This function was suggested but not proven by our 1984 study with mouse ts85 cells [63, 64].)
The CDC34 discovery [66] opened up a very large field, as it became clear (in general terms right away and in detail over the next two decades) that proteolysis by the Ub system underlies biological periodicity, from cell cycle oscillators to circadian rhythm oscillators. Other links between the Ub system and the cell cycle, including the 1991 demonstrations, that cyclins are degraded by the Ub system [220, 221], were identified after the above 1987-88 discoveries with RAD6 and CDC34 [65, 66].
Discovery of the first degradation signals (degrons) in short lived proteins and the first N‑degron pathway
Primary degradation signals are features (any features) of proteins that make them short-lived in vivo. In most settings, Ub itself is a "secondary" signal for proteolysis, in that Ub (or rather a poly-Ub chain; see below) is conjugated to a protein that contains a primary degradation signal. The nature of such signals was a puzzle until 1986 and the invention of the Ub fusion technique (see below) [58]. Using Ub fusions, Andreas Bachmair, Finley and I discovered the first degradation signals that target proteins for ubiquitylation and proteolysis, including the set of signals that give rise to the N-end rule of protein degradation [58, 59]. (The N-end rule relates the in vivo half-life of a protein to the identity of its Nt-residue.)
In 1991, I proposed to call degradation signals and their N-terminal subset "degrons" and "N‑degrons", respectively [60]. In the field at large, it took more than a decade for "degrons" to become a standard shorthand for "degradation signals" [43]. By identifying the first "primary" degradation signals in short lived proteins, the discovery of N-degrons solved the fundamental problem of selectivity in the Ub system. The N-end rule pathway (renamed, in 2019, as the Arg/N-degron pathway [43]) (Figs. 3G and 4), was the first of two specific pathways of the Ub system to be discovered, years before a multitude of other Ub-mediated pathways came into view. The second pathway is described below.
The ubiquitin fusion degradation (UFD) pathway
The second pathway of the Ub system, termed the UFD (Ub fusion degradation) pathway, was discovered also in 1986, essentially in parallel with the first N-end rule (N-degron) pathway [58]. The UFD pathway was subsequently characterized by Erica Johnson and Bonnie Bartel, then graduate students in the lab [111, 112]. Substrates of this pathway include a subset of Ub fusions in which the N-terminal Ub moiety cannot be cleaved off in vivo by deubiquitylases (DUBs), either at all or rapidly enough [58, 111, 112].
An impediment to cleavage by DUB enzymes can be a Pro residue at the Ub-protein junction (the presence of a junctional Pro residue slows down but does preclude the removal, by DUBs, of the Nt-Ub moiety from a Ub fusion) [58]. In contrast, an alteration of the last residue of the Ub moiety, e.g., the change of wildtype Nt-Gly-76 of Ub to Ala-76, completely precludes the cleavage by DUBs. We have found that a permanent and even transient retention of the Nt-Ub moiety in a Ub fusion results in the recognition of that moiety as a primary degradation signal by UFD4, the main Ub ligase of the UFD pathway [43, 111, 112, 222]. Detailed studies of the UFD pathway, particularly by the late Stefan Jentsch and colleagues (e.g., [222]), became an active and important field.
The Leu/N-degron pathway in bacteria
By the late 1980s, the functional and mechanistic understanding of the eukaryotic Arg/N-degron pathway (Figs. 3G and 4) became a major focus of my lab. (From now on, I shall use the 2019 "N-degron pathway" term [43], instead of the earlier term "N-end rule pathway"). In 1991, John Tobias (then a graduate student), Thomas Shrader (then a postdoc) and I extended the use of the Ub fusion technique [58] to prokaryotes, through the expression of Ubp1, a specific yeast DUB enzyme, in E. coli [110]. The resulting E. coli cells became capable of deubiquitylating an expressed Ub fusion. (Bacteria contain Ub-like proteins but lack a bona fide Ub system.)
We discovered that although E. coli lacked Ub, it did contain a distinct (Ub-independent) N‑degron pathway [110]. This bacterial proteolytic system is now called the Leu/N-degron pathway (Fig. 3D). Our lab's studies of this pathway identified, in particular, specific bacterial aminoacyl-tRNA-protein transferases (aa-transferases), encoded by the Aat and Bpt genes (the latter gene is absent in E. coli but is present in, e.g., Vibrio cholera and Vibrio vulnificus, as was shown in 2006 by Emmanuelle Graciet (then a postdoc in our lab). These aa-transferases are components of the Leu/N-degron pathway (Fig. 3D) [110, 114, 120].
The existence of a distinct N-degron pathway in bacteria, which lack a bona fide Ub system, suggests that N-degrons predated the Ub system. These and analogous evolutionary trajectories remain to be better understood. Mechanistic and functional studies of the bacterial Leu/N-degron pathway (Fig. 3D) are now a significant and dynamic field, with ongoing contributions by the Groisman, Bukau, Baker, Sauer, Dougan, Maurizi and other laboratories [167, 178, 182, 223-233].
Mechanistic determinants of degrons
In 1989, Bachmair and I carried out the first dissection of a degradation signal, and found that a eukaryotic N-degron (Arg/N-degron, in the modern terminology [43]) consists of at least three determinants: a destabilizing Nt-residue of a protein substrate (Figs. 3G and 4); at least one of substrate's internal Lys residues (the site of formation of a substrate-linked poly-Ub chain); and a conformationally disordered region [59]. As shown by Andreas Matouschek and colleagues, a disordered region functions as the site of initiation of processive degradation of a targeted substrate by the 26S proteasome [166, 234, 235].
In 1999, Tetsuro Suzuki (then a postdoc) and I advanced the analysis of N-degrons by identifying new such degrons through a novel genetic screen in a two-residue (Lys/Asn) sequence space. We found that an N-degron can consist of compositionally simple sequences that contain solely the Asn and Lys residues [115].
The multi-determinant organization of N-degrons recurs in other, subsequently identified degradation signals. Key differences among degrons include their first determinant, which is recognized by a specific E3 Ub ligase. In an N-degron, this determinant is a destabilizing Nt‑residue (Figs. 3G and 4), whereas in another degron it can be, for example, an internal (or C‑terminal) sequence motif. By revealing the basis of specificity of the intracellular protein degradation, the 1986 discovery of N-degrons and UFD degrons [58] has founded the field of degradation signals. This is a major arena nowadays, as hundreds of distinct, often conditional degrons in a multitude of cellular proteins are targeted by specific and regulated E3 Ub ligases of the Ub system.
N-terminal arginylation as a part of the Arg/N-degron pathway
In the early 1970s, Kaji and Soffer [236, 237] discovered a new reaction, the N-terminal arginylation of proteins, by the enzyme Arg-tRNA-protein transferase (R-transferase). In our 1986 paper about the first degrons in short lived proteins, we proposed that R-transferase and Nt‑arginylation are a part of the N-end rule pathway (called, at present, the Arg/N-degron pathway) [58] (Fig. 3G and 4). This hypothesis pinpointed the previously enigmatic biological function of R-transferases, identifying them as components of a specific proteolytic system [58]. Both the 1987 study by Ferber and Ciechanover [238] and our later work [106] confirmed the 1986 conjecture [58]. In 1990, Mordechai Choder (then a postdoc) collaborated with the laboratory of the late Andrea Goffeau to identify and clone the yeast Ate1 R-transferase [211]. More recent work with mammalian R-transferases is summarized below.
The first nonproteolytic function of ubiquitin
In 1987, Özkaynak, Finley and I cloned S. cerevisiae UBI1-UBI3, i.e., the Ub genes other than the (stress-specific) poly-Ub gene UBI4 [71]. In 1989, Finley, Bartel and I identified the nature of non-Ub parts of the UBI1-UBI3 proteins, and discovered a new function of Ub in this setting. UBI1-UBI3 were found to encode fusions of Ub to one protein of the large ribosomal subunit and one protein of the small ribosomal subunit, an arrangement conserved from yeast to mammals [68].
Remarkably, genetic analyses with precursors of both Ub and ribosomal proteins encoded by UBI1-UBI3 indicated that the presence of Ub in front of a specific ribosomal protein, despite being transient in vivo (the Ub moiety of a Ub fusion is removed by DUB enzymes), is required for efficient biogenesis of ribosomes [68]. In addition to discovering a specific role of the Ub system in the translation apparatus and protein synthesis, these results showed that in certain contexts Ub acts not as a degradation signal but as a cotranslational chaperone. This first nonproteolytic function of Ub [68] appeared to be an exceptional case until years later, when Linda Hicke and Howard Riezman demonstrated that ubiquitylation of an integral membrane protein signals its endocytosis [239]. Ub is now recognized to have numerous functions that are not directly proteolytic.
The first substrate-linked polyubiquitin chains
In 1989, using new approaches that included two-dimensional protein mapping assays, Vincent Chau and colleagues in my lab demonstrated that in vivo ubiquitylation of a short-lived protein substrate produces a substrate-linked poly-Ub chain of specific topology, with the isopeptide bonds between adjacent Ub moieties through the Lys-48 residue of Ub [77]. In contrast to linear precursors of Ub that are encoded by poly-Ub genes such as UBI4, a posttranslational poly-Ub chain is based on repeating, branch-generating isopeptide bonds between the adjacent Ub moieties in a chain.
This 1989 study also demonstrated that a poly-Ub chain linked to a substrate is essential for the substrate's degradation in vivo [77]. We proposed that a major function of substrate-linked poly‑Ub chains is to bind targeted substrates to the proteasome [77], a hypothesis confirmed by others, in part through the identification of poly-Ub binding proteins as components of the 26S proteasome. Later work by other laboratories has shown that poly-Ub chains can also be of different "local" topologies, with adjacent Ub moieties in a chain linked through Lys residues of Ub other than Lys-48. Some of these "alternative" poly-Ub chains have nonproteolytic functions [240]. The 1989 discovery of the first specific poly-Ub chains [77] illuminated the mechanistic role of Ub in proteolysis, and opened up yet another field of Ub studies.
The first physiological substrate of the ubiquitin system
In 1990, Mark Hochstrasser (then a postdoc) and I identified the first short-lived physiological substrate of the Ub system, the S. cerevisiae transcriptional repressor MATalpha2, and delineated its degradation signals [69, 212]. The 1990‑91 MATalpha2 papers were also the first direct evidence for the involvement of the Ub system in transcriptional regulation. A multitude of specific functions of Ub-dependent processes in transcription and its control have been discovered since 1990, including pathways that target hundreds of transcription factors in yeast, mammalian, and plant cells for regulated degradation ([143, 144, 241] and references therein).
The 1990-1991 MATalpha2 studies [69, 212] were also the beginning of insightful independent studies by Hochstrasser and colleagues that employed MATalpha2 as a fulcrum to dissect specific aspects of the Ub system. A mitotic cyclin was the second physiological substrate of this system to be characterized, in 1991, by the Kirschner and Hershko laboratories [220, 221]. Today, we know hundreds of such substrates. Moreover, it has become clear that most cellular proteins, including those that travel through or reside in membrane-enclosed compartments, can become physiological substrates of the Ub system at specific stages of their in vivo existence.
Discovery of the subunit selectivity of proteolysis by the ubiquitin system
In 1990, Erica Johnson, David Gonda and I discovered that the Ub system exhibits subunit selectivity, i.e., the ability to destroy a specific subunit of a protein, leaving the rest of the protein intact and thereby making possible protein remodeling [78]. Also in 1990, Hochstrasser and I detected subunit selectivity in the degradation of the MATalpha2 repressor, the first physiological substrate of the Ub system [69].
Subunit-selective proteolysis is a critical property of the Ub system, a feature both powerful and flexible, in that it enables protein degradation to be wielded as an instrument of protein remodeling for either positive or negative regulation. Through its ability to make protein machines compositionally dynamic, the subunit selectivity of degradation [69, 78] literally enables the vast physiology of Ub pathways. Many known examples of subunit selectivity include the degradation of cyclin subunits of cyclin-dependent kinases that spares their catalytic subunits; subunit-selective proteolytic activations of transcription factors, e.g., of NF-kappaB; and the subunit-selective degradation of a separase-produced Ct-fragment of a cohesin subunit by the Arg/N-degron pathway, a step that underlies the high fidelity of chromosome segregation (see above for a more detailed description of the latter process) [44].
Identifying and cloning the first specific E3 ubiquitin ligase
As mentioned above, in 1990, Bartel, Wünning and I employed genetic and biochemical approaches to identify, clone and analyze the first specific E3 Ub ligase, termed UBR1 [74]. This multifunctional 225 kDa protein is the sole E3 of the S. cerevisiae Arg/N‑degron pathway (Fig. 3G). (The term "Ub ligase" denotes either an E2-E3 holoenzyme or its E3 component.) This advance [74] opened up a particularly large field, as the human genome is now known to encode approximately 800 distinct Ub ligases. Through their regulated targeting of specific degrons in conditionally or constitutively short-lived proteins, these E3s underlie the immense functional reach of the Ub system.
Studies by many excellent laboratories that entered the Ub field in the 1990s and afterward have uncovered, among other things, the existence of more than ten Ub-like conjugation pathways. These pathways involve Ub-like proteins (called SUMO, NEDD8, etc.) and specific enzymes that are similar to the enzymes of Ub conjugation. Ub-like pathways have a number of functions, mostly (though not exclusively) nonproteolytic ones. These pathways interact with the Ub system, the largest one and the first to be characterized. The resulting "meta-set" of the Ub and Ub-like systems has a strikingly broad functional range and mechanistic diversity [76, 242-253].
Ramifications of the early advances
During the 1980s, two complementary advances, the in vitro mechanistic discovery of the Ub‑dependent proteolysis by the Hershko laboratory and our discovery of degrons and physiological fundamentals of the Ub system [43, 45, 48], have produced, together, a novel view of cellular physiology and a novel approach to biological studies.
In that new understanding, the regulated intracellular proteolysis became tremendously more important than it was thought to be four decades ago. These advances, together with later studies by many groups, including our laboratory, have shown that the control through regulated protein degradation rivals, and often surpasses in significance, the classical regulation through transcription and translation. This understanding of the logic of biological circuits is of major relevance for medicine, given the immense functional range of the Ub system and the multitude of ways in which Ub-mediated processes can malfunction in disease, from cancer and neurodegeneration to perturbations of immunity and many other illnesses, including "normal" aging and birth defects.
Pharmaceutical companies and academic laboratories are developing compounds that target specific components of the Ub system. The fruits of their labors have already become, or will soon become, clinically useful drugs. Work in this arena is producing not only "conventional" inhibitors or activators of enzymes but also drugs that would direct a Ub ligase system to target, destroy and thereby downregulate any specific protein. Given the broad functional range of N‑degron (Figs. 3 and 4) and C-degron pathways, they will be a part of coming medical advances.
What follows are brief descriptions of other, post-1990 contributions by our laboratory, including Ub studies.
Sequelog and spalog, for denoting sequence and spatial similarities
In 2004, I proposed the term sequelog [193], to address a stark disconnect between the relative rigor of statistical methods for computing similarities among amino acid sequences (or nucleotide sequences) and the often non-rigorous use of the terms "homolog", "ortholog" and "paralog". "Sequelog" denotes an amino acid or nucleotide sequence that is similar, to a specified extent, to another sequence. Derivatives of sequelog include "sequelogy" (sequence similarity) and "sequelogous" (similar in sequence).
The usefulness of sequelog and derivative notations stems from the rigor and clarity of their evolutionary and functional neutrality. By contrast, in settings that use "homolog," "ortholog," and "paralog" (they denote, respectively, common descent and functional similarity/dissimilarity), these terms are often interpretation-laden and can be quite imprecise [193]. "Homolog", "ortholog", and "paralog" are compatible with the sequelog terminology. The former terms can be used to convey understanding about common descent and biological functions, if this additional information, distinct from sequelogy per se, is actually present.
The second term, proposed in the same brief paper, was "spalog". It denotes a 3-D structure that is similar, to a specified extent, to another 3-D structure. In addition to filling a lacuna in the existing terminology for 3-D structures, a major advantage of "spalog" (analogously to "sequelog") is its evolutionary and functional neutrality [193].
Later studies of N-degron pathways and related subjects
Our post-1990 studies concentrated on N-degron pathways (previously called "N-end rule pathways" [43]). Until 2010, there were only two of them, the eukaryotic one (it is called, at present, the Arg/N-degron pathway; Figs. 3G and 4), and the bacterial one (it is called, at present, the Leu/N-degron pathway; Fig. 3D). Several other N-degron pathways were discovered in 2010-2018 (Fig. 3). We have been studying N-degron pathways in fungi (S. cerevisiae), mammals (human cells and the mouse M. musculus), and in prokaryotes such as E. coli and the human pathogen Vibrio vulnificus.
What follows is a summary of some contributions by the laboratory to the Ub field over the last ~25 years, from the mid-1990s to 2021.
The Arg/N-degron pathway is universal among eukaryotes. It targets proteins for degradation by recognizing, in particular, their specific destabilizing Nt-residues. Among them, the Nt-Asn and Nt-Gln residues are destabilizing owing to Nt-amidases. In the yeast S. cerevisiae, the NtN,Q‑amidase Nta1 deamidates either Nt-Asn or Nt-Gln to, respectively, Asp or Glu. In multicellular eukaryotes, the NtN-amidase Ntan1 deamidates Nt-Asn, while the NtQ‑amidase Ntaq1 deamidates Nt-Gln, yielding proteins that bear Nt-Asp or Nt-Glu. These Nt-residues are Nt‑arginylated by the Ate1 R-transferase. The resulting Nt-Arg is recognized by specific ubiquitin ligases and/or by the p62 protein, and thereby causes degradation of Nt-Arg-bearing proteins by the proteasome and/or autophagy. N-recognins, specifically N-degron-recognizing E3s Ub ligases of the Arg/N‑degron pathway, are Ubr1 in S. cerevisiae and Ubr1, Ubr2, Ubr4 and Ubr5 in mammals and other animals (Figs. 3G and 4 and references therein).
As described above, the 225-kDa S. cerevisiae Ubr1 E3 was the first cloned E3 Ub ligase, in 1990, by Bartel and Wünning in our lab (Fig. 3G) [74]. In 1998, Yong Tae Kwon (then a postdoc in the lab) and colleagues have identified and cloned the mouse and human genes encoding Ubr1 and Ubr2, two sequelogous (similar in sequence [193]) N-recognin E3s of the human/mouse Arg/N-degron pathway (Fig. 4) [75].
Discovery that Cdc48, an abundant ATPase, is required for protein degradation
In 1996, Michel Ghislain, Jürgen Dohmen and Frédéric Lévy (then postdocs in the lab) have shown, as a part of their study of the S. cerevisiae Arg/N-degron and UFD pathways, that Cdc48, an abundant multisubunit ATPase, is essential for the proteasome-mediated degradation of some among our test proteins [254]. This discovery was the first glimpse of the central importance of Cdc48, a "segregase" (as it was later termed), in many proteolytic pathways of the Ub system in both yeast and multicellular eukaryotes.
Later studies by other labs have revealed a remarkably broad functional range of Cdc48. Most of its functions have in common the ability of the Cdc48 ATPase to unfold proteins (including polyubiquitylated proteins) by pulling the targeted polypeptides through the central pore of the Cdc48 homohexamer [255-257].
Cloning and analyses of Nta1, the Nt-amidase of the S. cerevisiae Arg/N-degron pathway
As mentioned above, the S. cerevisiae Nta1 NtN,Q-amidase can deamidate either Nt-Asn or Nt‑Gln (Fig. 3G). Nta1 was identified and cloned in our lab by Rohan Baker (then a postdoc) in 1995 [108]. Its 3-D crystal structure was determined in 2016 by Hyun-Kyu Song and colleagues [199].
Unexpectedly, the S. cerevisiae Nta1 NtN,Q-amidase was found to be located largely (>80%) in the mitochondrial inner matrix, where it might be a part of a still hypothetical mitochondrial N‑degron pathway (nothing definitive is known about this possibility). The cytosolic/nuclear pool of Nta1 (<20% of total) is the one that mediates the deamidation branch of the yeast Arg/N‑degron pathway (Fig. 3G). The single NTA1 gene encodes both mitochondrial and cytosolic versions of NtN,Q-amidase, apparently through a differential use of two specific "upstream" and "downstream" AUG (Met) codons for translation initiation [108].
Although the Nta1 NtN,Q-amidase was cloned nearly three decades ago [108], its physiological substrates in S. cerevisiae are still unknown. The same is true of the S. cerevisiae Ate1 R‑transferase (arginyltransferase) (Fig. 3G) (its identification and cloning were described above [211]), in contrast to R-transferases of multicellular eukaryotes (in animals and plants) (Fig. 4), whose many known physiological substrates mediate the functions of the Arg/N-degron pathway in, for example, the sensing of oxygen and nitric oxide.
Our analyses, in 1995, of specific effects of Nta1 overexpression, in S. cerevisiae, on the rates of degradation of engineered Arg/N-degron substrates suggested (but did not prove) the existence of a targeting complex that contained (at least) the Nta1 NtN,Q-amidase, the Ate1 R-transferase, and the Ubr1‑Rad6 E3-E2 Ub ligase [108]. In 2020, Jang-Hyun Oh (a postdoc in the lab) and his colleagues did identify such a complex, using, in particular, two-hybrid (Y2H) binding assays [142]. In addition to the above components (Nta1, Ate1, and Ubr1-Rad6 E3-E2), this complex was also found to contain the second (Ubr1‑bound) E3-E2 Ub ligase, Ufd4-Ubc4/5 [142].
The involvement of Ufd4 in the S. cerevisiae Arg/N-degron pathway was discovered in 2010, by Cheol-Sang Hwang (then a postdoc in the lab) and Anna Shemorry (then a graduate student) [126]. They identified and analyzed Ufd4 as a part of the functionally relevant double-E3 Ubr1‑Rad6/Ufd4-Ubc4/5 complex [126] (see below).
Cloning and analyses of Ntan1, the NtN-amidase of the Arg/N‑degron pathway in multicellular eukaryotes
The Ntan1 NtN-amidase, one mammalian counterpart of the yeast Nta1 Nt-amidase, was cloned in 1996, by Sergey Grigoryev (then a postdoc in our lab) and his colleagues (Fig. 4) [113]. In contrast to the S. cerevisiae Nta1 Nt-amidase (Fig. 3G), the Nt-deamidation activity of Ntan1 is confined to Nt-Asn. In addition, unlike the largely mitochondrial yeast Nta1 NtN,Q-amidase (Fig. 3G), the mammalian Ntan1 NtN-amidase and Ntaq1 NtQ-amidase (Fig. 4) are present largely in the cytosol/nucleus [125].
In the fly D. melanogaster, the cleavage, by a caspase, of the antiapoptotic Ub ligase Diap1 generates the short-lived Asn21-Diap1 Ct fragment that is much less efficacious (owing to its rapid degradation) than full-length Diap1 in repressing apoptosis, as has been shown in 2003 by the laboratory of Pascal Meyer [258]. Asn21-Diap1 bears Nt-Asn. Consequently, its degradation by the Arg/N-degron pathway requires the Ntan1 NtN-amidase [258].
A virus would benefit from a delay of apoptosis, as this would facilitate the completion of viral replication in an infected cell. It was found, by Wang et al. [259], that a picorno-like RNA virus, induces, through a currently unknown mechanism, the proteasome-dependent degradation of the Ntan1 NtN-amidase in infected insect cells, resulting in a partial stabilization (upregulation) of the Asn21-Diap1 Ct-fragment. Thus, through a down-regulation of Ntan1, a virus can inhibit apoptosis of infected cell, thereby benefiting the infection cycle [259].
Cloning and analyses of Ntaq1, the NtQ-amidase of the Arg/N-degron pathway in multicellular eukaryotes
The Ntaq1 NtQ-amidase, the second, "complementary" Nt-amidase of the mammalian Arg/N‑degron pathway, selectively deamidates Nt-Gln. Mouse Ntaq1 was purified and cloned in 2009, more than a decade after Ntan1, by Haiqing Wang, Konstantin Piatkov and Christopher Brower (then postdocs in the lab) [125]. Both the 3-D crystal structure of the Ntaq1 NtQ-amidase and its analyses by site-directed mutagenesis indicated that its active site and catalytic mechanism are similar to those of transglutaminases [125, 260].
Usp1 is one of about 100 distinct deubiquitylases (DUB enzymes) encoded by a mammalian genome. Usp1 forms a heterodimeric complex with Uaf1, a "scaffold" protein. The binding of Uaf1 to Usp1 strongly increases the DUB activity of Usp1 [261]. In addition to being a "conventional" DUB, Usp1 can also cleave itself, immediately after the internal Gly-Gly sequence, which is identical to the C-terminal Gly-Gly sequence of Ub [262]. The resulting Ct-fragment of Usp1, denoted as Gln-Usp1Ct, bears Nt-Gln and contains a part of the sequence (‘‘His box'') that encompasses the active site of Usp1 [262]. Remarkably, the DUB activity of the autocleaved Usp1 can be retained, since the Usp1Nt Nt-fragment and the Gln‑Usp1Ct Ct-fragment can be transiently held together within the autocleaved Usp1-Uaf1 complex [263].
In 2012, Piatkov in our lab, in a collaboration with the laboratory of Tony Huang, has identified the Gln-Usp1Ct Ct-fragment of the autocleaved Usp1 as the first physiological substrate of the Arg/N‑degron pathway (Fig. 4) that is targeted for degradation through the deamidation of Nt‑Gln, a reaction mediated by the Ntaq1-encoded NtQ-amidase [129]. It was also found that a metabolic stabilization of the Gln-Usp1Ct Ct-fragment results in mammalian cells that are hypersensitive to UV irradiation [129]. Thus, in addition to its other functions in DNA damage responses and chromosome segregation, the Arg/N-degron pathway (Fig. 4) regulates genomic stability through the Ntaq1-mediated conditional degradation of the autocleaved Usp1 deubiquitylase. More recent studies, particularly the one by the Huang laboratory [264], greatly advanced the understanding of the Usp1 autocleavage, the Ntaq1-dependent instability of the resulting Gln-Usp1Ct Ct-fragment, and the Usp1-mediated regulation of DNA replication forks under both normal and stressful conditions.
Other studies of Ntan1 and Ntaq1
In 2000, Yong Tae Kwon (then a postdoc) and colleagues in the lab constructed Ntan1-/- mouse strains that lacked the Ntan1 NtN-amidase [187]. In 2012, Piatkov and colleagues in the lab constructed Ntaq1-/- mouse strains that lacked the Ntan1 NtQ-amidase [129]. Both of these mutant mouse strains were viable, fertile, and not overtly abnormal [129, 187]. At least Ntan1-/- mice appeared to exhibit abnormalities in learning and memory [187].
Some physiological protein substrates of Ntan1 and Ntaq1 have been identified, and many other substrates are predicted to exist as well (e.g., [43]). Studies by the laboratories of Emmanuelle Graciet and Michael Holdsworth, with plant mutants of Arabidopsis thaliana that lack either Ntan1 or Ntaq1, indicated the importance of these Nt-amidases (in ways that remain to be mechanistically understood) for immune responses by plants [265, 266].
In her unpublished study, Xia Wu (then a postdoc in our lab) carried out heterozygous matings of single-mutant Ntan1‑/‑ and Ntaq1-/- mice that yielded a double-mutant [Ntan1‑/‑ Ntaq1‑/‑] mouse strain whose cells lacked both NtN-amidase and NtQ‑amidase, and therefore could not deamidate either Nt-Asn or Nt-Gln. Nevertheless, these double-mutant [Ntan1‑/‑ Ntaq1-/-] mice, similarly to single-mutant Ntan1‑/‑ and Ntaq1-/- mice, were found to be fertile and not overtly abnormal (X. Wu and A.V., unpublished data). While the Ntan1 and Ntaq1 Nt-amidases play a role at least in immunity and neural functions, the molecular mechanisms of these functions and most of cognate Nt-amidase substrates remain to be identified. An exception is the already known necessity of the Ntaq1 NtQ-amidase for the degradation of the Gln-Usp1Ct Ct-fragment (see above and [129, 264]).
The fact that physiological roles of the mouse Ntan1 NtN-amidase and Ntaq1 NtQ-amidase are relatively inconspicuous (under laboratory conditions) is in striking contrast to major phenotypic consequences (including lethality) of losing either the Nt-arginylation branch (via the Ate1 R‑transferase) or the ubiquitylation branch (via N-recognin Ub ligases) of the mammalian Arg/N-degron pathway (Fig. 4) [43, 118, 194, 195].
Interestingly, there are no significant sequelogies (sequence similarities [193]) between the yeast Nta1 NtN,Q-amidase, the mammalian mouse Ntan1 NtQ-amidase and the mammalian Ntaq1 NtQ‑amidase, despite close similarities of their Nt-deamidation reactions [108, 113, 125, 199]. This and other evidence strongly suggests that the three Nt-amidases have evolved independently from one another. Specifically, it is likely that an Arg/N-degron pathway in the last common ancestor of fungi/yeasts, animals and plants has "acquired" the Nt-deamidation step during a later evolution of this pathway in diverging organismal lineages. Note that the Ubr1 N-recognin (E3 Ub ligase) and the Ate1 R-transferase of the Arg/N-degron pathway (Figs. 3G and Fig. 4) are conserved among fungi/yeasts, animals and plants, in contrast to Nt-amidases.
N-terminal arginylation as a part of the Arg/N-degron pathway
In the 1986 study that discovered the first degrons (N-degrons) in short-lived proteins, we proposed that the previously identified R-transferase and Nt-arginylation (their biological function was unknown at the time) are a part of biochemical circuits that underlie the activity of N-degrons [58] (Fig. 3G and 4). This conjecture was confirmed [106, 238].
The 60-kDa Ate1 R-transferase catalyzes the conjugation of Arg (provided by Arg-tRNA) to the α-amino group of a specific Nt-residue of a protein (Nt-Asp, Nt-Glu, or oxidized Nt-Cys* (Cys‑sulfinate or Cys-sulfonate). The resulting Nt-Arg can be bound by Arg/N-recognins (Figs. 3G and 4). In mammals, there are six isoforms of R-transferase, produced through alternative splicing of the Ate1 pre-mRNA [267, 268]. Many natural cleavage-generated Ct‑fragments of specific proteins, are either confirmed or putative short-lived substrates of the Ate1 R-transferase and the rest of the Arg/N-degron pathway [42, 43, 168].
In 1999, Yong Tae Kwon and Anna Kashina (then postdocs in our lab) cloned the mouse Ate1 R-transferase (Fig. 4), which was found to be highly sequelogous to its S. cerevisiae counterpart [267]. In 2002, Kwon and colleagues constructed an unconditional Ate1-/- mouse strain, which lacked the Ate1 R-transferase and was found to be an embryonic lethal [117]. Ate1-/- embryos die in mid-gestation with defects in heart development and in angiogenic remodeling of the early vascular plexus [117].
In 2009, Christopher Brower (then a postdoc in the lab) used the Cre-lox technique to delete the mouse Ate1 gene conditionally (in adult mice), since the unconditional Ate1 deletion has been shown to be lethal (see above) [124]. Brower found that a postnatal deletion of the Ate1 R‑transferase causes a rapid decrease of body weight and results in early death of ~15% of Ate1‑deficient mice. Surviving Ate1-deficient mice contained little visceral fat, despite being hyperphagic. They also exhibited an increased metabolic rate, ectopic induction of the Ucp1 uncoupling protein in white fat, and were resistant to diet-induced obesity. In addition, Ate1‑deficient mice had enlarged brains, an enhanced startle response, and were also strikingly hyperkinetic, as well as prone to seizures and kyphosis. Ate1-deficient males were also infertile, owing to defects in Ate1-/- spermatocytes [124]. The remarkably broad range of biological processes that are perturbed by the loss of Nt-arginylation is consistent with a large (and growing) number of mammalian physiological Ate1 substrates that have been identified (or inferred) over the last two decades.
Recently, the laboratory of Yong Tae Kwon discovered that p62, a component of the autophagy-lysosome system, is also a non-E3 Arg/N-recognin that binds to cytosolic proteins which bear, in particular, Nt-Arg. p62 mediates the capture of these proteins by autophagy and their subsequent destruction in lysosomes [146, 147, 157, 269]. BiP (an Hsp70 chaperone that resides in the ER), calreticulin (another ER chaperone), and protein disulfide isomerase are among ER-resident proteins that bear Nt-arginylatable Nt-residues such as Nt-Asp or Nt-Glu. Upon stresses, including heat shock and/or unfolded protein response (UPR), a fraction of these ER proteins is transferred to the cytosol, followed by their Nt-arginylation and degradation by the autophagy-lysosome system, and also by the 26S proteasome [146, 147]. In sum, the Arg/N-degron pathway was found, by Kwon and his colleagues, to be a functional link between UPS and autophagy.
Arginylation and the sensing of oxygen and nitric oxide
In 2005, Rong-Gui Hu (then a postdoc in our lab) and his colleagues, and also, independently, the laboratory of Yong Tae Kwon discovered that the mammalian Arg/N-degron pathway is a new kind of oxygen (O2) and nitric oxide (NO) sensor. The NO/O2-dependent oxidation of Nt‑Cys converts it to Nt-Cys-sulfinate or Nt-Cys-sulfonate, which can be Nt-arginylated, in contrast to unmodified Nt-Cys [119, 270]. The NO/O2‑dependent proteolysis by the Arg/N‑degron pathway controls the levels of some proteins that bear Nt-Cys, including Rgs4, Rgs5 and Rgs16 [119, 270]. These conditionally short-lived proteins are regulators of specific G proteins.
The Arg/N‑degron pathway is also the main sensor of NO/O2 in plants, through the NO/O2‑dependent oxidation of Nt-Cys in conditionally short-lived transcription factors that include Rap2.12, Rap2.2, Rap2.3, Hre1, and Hre2 [171, 179, 181, 271]. Both in plants and animals, the NO/O2-dependent oxidation of Nt-Cys is catalyzed by Cys-oxidases, in addition to a nonenzymatic oxidation of Nt-Cys. In vivo levels of the above transcription factors and the expression of regulons controlled by them underlie adaptations to a broad range of stresses in animals and plants [170, 171, 179, 181, 271].
The Arg/N-degron pathway as a sensor of heme
In 2008, Rong-Gui Hu and colleagues in our lab discovered that mammalian and S. cerevisiae Ate1 R-transferases are inhibited by low-μM levels of hemin (Fe3+-heme) [121]. Hemin also accelerates, in vivo, the degradation of mouse Ate1, thereby acting as both a "stoichiometric" and "catalytic" down-regulator of Nt-arginylation. Thus, in addition to being a sensor of NO, O2 and short peptides, the Arg/N-degron pathway is also a sensor of heme [121]. One function of the Arg/N-degron pathway may be to coordinate the activities of protein effectors, both reacting to and controlling the redox dynamic of heme, oxygen, NO and thiols, in part through the conditional degradation of specific transcriptional regulators and regulators of G proteins, such as Rgs4, Rgs5 and Rgs16 [119, 121].
Identification of a protein, termed Liat1, that binds to the Ate1 arginyltransferase and contains tandem 10-residue repeats
In 2014, Christopher Brower and colleagues in our lab discovered a previously uncharacterized mouse protein, termed Liat1 (ligand of Ate1), that physically interacted with the mouse Ate1 R‑transferase, without being a substrate of Ate1 [272]. Liat1 stimulated the in vitro N-terminal arginylation of a model substrate by Ate1. All examined vertebrate and some invertebrate genomes encode proteins sequelogous (similar in sequence) to mouse Liat1. Sequelogs of Liat1 share a highly conserved ∼30-residue region that has been shown, by Brower and colleagues, to be required for the binding of Liat1 to Ate1 [272]. In contrast to Liat1 genes of nonprimate mammals, Liat1 genes of primates (including humans) are subtelomeric, a location that tends to confer evolutionary instability on a gene. Remarkably, Liat1 proteins of some primates, from macaques to humans, were found to contain tandem repeats of a 10-residue sequence, whereas Liat1 proteins of other mammals contained a single copy of this motif [272].
Later work by Christopher Brower, in his own laboratory, has shown that the N-terminal half of Liat1 comprises an intrinsically disordered region (IDR) that facilitates its liquid-liquid phase separation (LLPS) in the nucleoli of mammalian cells [273]. Brower and colleagues also found that Liat1 is targeted to the nucleolus by a low-complexity poly-lysine region within its IDR. In addition the lysyl-hydroxylase activity of the enzyme Jmjd6 modifies Liat1 (in a manner that requires its poly-lysine poly-K region) and thereby inhibits its nucleolar targeting. In sum, this study, by the Brower laboratory, revealed that Liat1 participates in the nucleolar liquid-liquid phase separation, and is regulated by Jmjd6 [273]
Mgt1 and the discovery of double-E3 design of the Arg/N-degron pathway
Protein substrates of the S. cerevisiae Arg/N-degron pathway are recognized, in particular, through their Arg/N-degrons by the Ubr1 E3 Ub ligase (Figs. 3G and 4). In 2009, Cheol-Sang Hwang (then a postdoc in the lab) and Anna Shemorry (then a graduate student) discovered that Mgt1, a yeast DNA repair protein, is targeted for degradation by both the Ubr1 E3 and the Ufd4 E3, which recognize a non-N-terminal degron of Mgt1 [274]. Ufd4 is an E3 enzyme of the ubiquitin-fusion degradation (UFD) pathway that recognizes an N-terminal ubiquitin moiety. In their 2010 study, Hwang and Shemorry discovered that the RING-type Ubr1 E3 and the HECT‑type Ufd4 E3 interact, both physically and functionally [126]. Although Ubr1 can recognize and polyubiquitylate an Arg/N-degron substrate in the absence of Ufd4 (Ufd4 is not an N-recognin), the double-E3-E2 Ubr1-Rad6/Ufd4-Ubc4/5 complex is more processive, in that it produces a longer substrate-linked polyubiquitin chain. Conversely, Ubr1 was found to function as a polyubiquitylation-enhancing component of the Ubr1-Ufd4 complex in its targeting of UFD substrates [126]. It was also found that Ubr1 can recognize the N-terminal ubiquitin moiety. These and related advances in 2010 [126] unified two proteolytic systems that have been studied separately for more than two decades.
Johanson-Blizzard syndrome and the human Ubr1 E3 N-recognin
In 2005, Martin Zenker, a medical geneticist in Germany, led a collaborative study that included our lab. This work identified homozygous inactivating mutations in the UBR1 gene as the cause of Johanson-Blizzard syndrome (JBS) [127, 275]. Children with JBS lack the Ubr1 Arg/N‑recognin but retain other Arg/N‑recognins, including Ubr2, a sequelog [193] and functional analog of Ubr1 (Fig. 4). Symptoms of JBS include an exocrine pancreatic insufficiency and inflammation, multiple malformations (e.g., a near-absence of nasal wings), as well as mental retardation and deafness ([42, 275] and references therein). Ubr1-/- mice exhibit JBS symptoms in a milder form. Mice lacking Ubr2 have other defects, including infertility in males (owing to apoptosis of spermatocytes) and genomic instability [168].
Accelerators of apoptosis as Arg/N-degron substrates
During apoptosis, caspases cleave nearly 2,000 different proteins in a mammalian cell. Caspase‑mediated cleavages of cellular proteins can generate proapoptotic Ct-fragments, defined as those that increase the probability of apoptosis. Such Ct-fragments often bear destabilizing Nt‑residues. In 2012, Konstantin Piatkov and Christopher Brower (then postdocs in the lab) have shown that the natural proapoptotic Ct-fragments Cys-Ripk1, Cys-Traf1, Asp-Brca1, Leu-Likk1, Tyr-Nedd9, Arg-Bid, Asp-BclXL, Arg-BimEL Asp-Epha4, and Tyr-Met were short-lived substrates of the Arg/N-degron pathway [128]. In agreement with these results, even a partial ablation of the Arg/N‑degron pathway sensitizes cells in culture to apoptosis [128]. In sum, the Arg/N‑degron pathway is a regulator of apoptosis, acting largely (but not necessarily exclusively) as an antiapoptotic circuit [128]. By destroying proapoptotic Ct-fragments, the Arg/N-degron pathway contributes to thresholds that prevent a transient or otherwise weak proapoptotic signal from reaching the point of commitment to apoptosis.
In 2013, Brower and Piatkov in our lab have shown that several natural proteins that are proteolysis-generated mammalian Ct-fragments that are either known or suspected to accelerate neurodegeneration (including Ct-fragments of Tau, Tdp43, and α-synuclein) are short-lived substrates of the Arg/N-degron pathway [173], suggesting that the down-regulation of such fragments by the Arg/N-degron pathway counteracts neurodegeneration.
Physical association of specific E3 ubiquitin ligases and the 26S proteasome
In 2000, Youming Xie (then a postdoc in the lab) discovered that both S. cerevisiae Ubr1, the E3 Ub ligase of the Arg/N-degron pathway (called, then, the "N-end rule pathway") and Ufd4, the E3 Ub ligase of the UFD pathway, interact with specific subunits of the 26S proteasome [276]. In addition to the possibility that these interactions underlie a route for delivery of ubiquitylated substrates to the proteasome, our results suggested that at least some Ub ligases may target their substrates while being bound to the 26S proteasome. Other groups reported that several other Ub ligases also interacted with the 26S proteasome. In 2002, Xie and I showed that a mutant S. cerevisiae UFD4 Ub ligase that lacked its proteasome-binding region could still mediate ubiquitylation of UFD substrates but their proteolysis was impaired, strongly suggesting that the proteasome-UFD4 interaction is functionally relevant [277].
Five enzymes of the Arg/N-degron pathway form a targeting complex: the concept of superchanneling
In 2020, Jang-Hyun Oh, Ju-Yeon Hyun, Stanley (Shun-Jia) Chen and I discovered the targeting complex of the Arg/N-degron pathway, in both the S. cerevisiae and human versions of this proteolytic system [142].
Specifically, the human Nt-Asn-specific Ntan1 NtN-amidase, the Gln-specific Ntaq1 NtQ‑amidase, the R-transferase (arginyltransferase) Ate1, and the E3-E2 Ub ligase Ubr1‑Ube2A/B (or Ubr2-Ube2A/B) form a complex in which the Ntan1 NtN-amidase binds to the Ntaq1 NtQ-amidase, to the Ate1 R-transferase, and to the Ubr1 (or Ubr2). In addition, the Ntaq1 NtQ-amidase and the Ate1 R-transferase also bind to Ubr1(or Ubr2). In S. cerevisiae, the Nta1 NtN,Q-amidase, the Ate1 R-transferase, and the double-E3-E2 Ub ligase complex Ubr1‑Rad6/Ufd4-Ubc4/5 were also found to form an analogous targeting complex [142].
These complexes may enable substrate channeling, in which a substrate bearing, for example, Nt-Asn, would be captured by a complex-bound Nt-amidase, followed by sequential Nt‑modifications of the substrate and its polyubiquitylation at an internal Lys residue without substrate's dissociation into the bulk solution. At least in yeast, the Ubr1/Ufd4 Ub ligase interacts with the 26S proteasome (see the above summary) [277], suggesting an even larger Arg/N-degron-targeting complex that contains the proteasome as well [142].
In addition, specific features of protein-sized Arg/N-degron substrates, including their partly sequential and partly nonsequential enzymatic modifications, led us to a new and verifiable concept termed "superchanneling". In superchanneling, the synthesis of a substrate-linked poly‑Ub chain can occur not only after a substrate's sequential Nt modifications, but also before them, through a skipping of either some or all of these modifications within a targeting complex [142].
Substrates of the Arg/N-degron pathway and their protection by chaperones
In 2017, Jang-Hyun Oh, Ju-Yeon Hyun and I found that the heat shock protein 90 (Hsp90) chaperone system of S. cerevisiae is greatly impaired in naa10Δ cells, which lack the NatA Nt‑acetylase and therefore cannot N-terminally acetylate a majority of normally Nt-acetylated proteins, including Hsp90 and most of its cochaperones [137].
Chk1, a mitotic checkpoint kinase and a client of Hsp90, was degraded relatively slowly in wild-type cells but was rapidly destroyed in naa10Δ cells by the Arg/N-end rule pathway, which recognized a C‑terminus-proximal degron of Chk1. We also found that in the absence of NatA Nt-acetylase, several diverse proteins (in addition to Chk1) become much shorter-lived, owing to their accelerated degradation by the Arg/N-degron pathway. These proteins include Kar4, Tup1, Gpd1, Ste11, and also, remarkably, the main Hsp90 chaperone (Hsc82) itself. Protection of Chk1 by Hsp90 could be overridden not only by ablation of the NatA Nt-acetylase but also by overexpression of the Arg/N-end rule pathway in wild-type cells. These and related results revealed a major role of Nt-acetylation in the Hsp90-mediated protein homeostasis, a strong up‑regulation of the Arg/N-end rule pathway in the absence of NatA, and also showed that a number of Hsp90 clients are previously unknown substrates of the Arg/N-end rule pathway [137].
The ATF3 transcription factor as a short-lived substrate of the Arg/N-degron pathway
In mammals, the Arg/N-degron pathway is mediated by the Ubr1, Ubr2, Ubr4, and Ubr5 E3 Ub ligases, and by the p62 regulator of autophagy. Ubr1 and Ubr2 are sequelogous, functionally overlapping, and dominate the targeting of Arg/N-degron substrates in examined cell lines.
In a study published in 2020, Tri Vu (then a postdoc in our lab) constructed mouse strains in which the double mutant [Ubr1-/- Ubr2-/-] genotype can be produced, conditionally, in adult mice. We also constructed human [Ubr1-/- Ubr2-/-] HEK293T cell lines that unconditionally lacked both Ubr1 and Ubr2 [143]. Atf3 is a basic leucine zipper transcription factor (TF) that regulates hundreds of genes and can act as either a repressor or activator of transcription. Using the above double-mutant mice and human cells, we found that the levels of endogenous, untagged Atf3 were significantly higher in both of these [Ubr1-/- Ubr2-/-] settings than in wildtype cells. We also found, through chase-degradation assays with [Ubr1-/- Ubr2-/-] and wildtype human cells, that the Arg/N-degron pathway mediates a large fraction of Atf3 degradation. In addition, we used split-Ub and another protein interaction assays to detect the physical binding of human Atf3 to both Ubr1 and Ubr2, in agreement with the demonstrated degradation of endogenous Atf3 by the Arg/N-degron pathway. The full-length 24 kDa Atf3 binds to ∼100 kDa fragments of 200 kDa Ubr1 and Ubr2 but does not bind (in the setting of interaction assays) to the full-length Ubr1/Ubr2. These and other binding patterns, whose mechanics and functional significance remain to be understood, indicate a conditional (regulated) degradation of Atf3 by the Arg/N-degron pathway [143].
The Arg/N-degron pathway targets transcription factors and regulates specific genes
The second 2020 study by Tri Vu [144] employed double-knockout (2-KO) [Ubr1-/- Ubr2-/-] human HEK293T human cell lines that were constructed in the first 2020 study by Vu (see above [143]). In the present study, a collaboration with Dylan Mitchell and Steven Gygi (Harvard Medical School, Boston, MA), we studied 2-KO) [Ubr1-/- Ubr2-/-] HEK293T cells in conjunction with RNA-sequencing, mass spectrometry (MS), and split-Ub binding assays. Summarized below are major results:
- Some mRNAs, such as those encoding lactate transporter Mct2 and β-adrenergic receptor Adrb2, are strongly (∼20-fold) up-regulated in 2-KO cells, whereas other mRNAs, including those encoding Magea6 (a regulator of Ub ligases) and Lcp1 (an actin-binding protein), are completely repressed in 2-KO cells, in contrast to wildtype cells.
- Glucocorticoid receptor (GR), an immunity-modulating transcription factor (TF), is upregulated in 2-KO cells. In addition, GR physically binds to Ubr1, strongly suggesting that GR is a physiological substrate of the Arg/N-degron pathway.
- Prep1, another TF, was also found to bind to Ubr1.
- MS-based analyses identified ∼160 proteins whose levels were increased or decreased by more than 2-fold in 2-KO cells. For example, the homeodomain TF Dach1 and the neurofilament subunits NF-L (Nfel) and NF-M (Nfem) were expressed in wildtype cells but were virtually absent in 2-KO cells.
- The disappearance of some proteins in 2-KO cells took place despite up-regulation of their mRNAs, suggesting that the Arg/N-degron pathway can also modulate translation of specific mRNAs.
In sum, the Arg/N-degron pathway was found to be a regulator of mammalian gene expression, in part through conditional targeting of TFs that include Atf3, GR, and Prep1 [144].
Identification of S. cerevisiae Cup9 as a transcriptional repressor that regulates the import of peptides and is targeted for degradation by Arg/N-degron pathway
Ptr2 is the main transmembrane importer of di/tripeptides in S. cerevisiae [278]. The laboratory of Jeffrey Becker carried out a genetic screen for yeast mutants that could not import peptides and discovered that one of their mutants was in the previously identified (by our lab) UBR1 gene that encodes the 225-kDa N-recognin E3 Ub ligase of Arg/N-degron pathway [49, 74].
Christopher Byrd and Glenn Turner (then graduate students in our lab) set out to identify the mechanism through which the Arg/N-degron pathway controls the import of peptides. They carried out a genetic screen with ubr1Δ S. cerevisiae that lacked Ubr1 and therefore could not import peptides. The aim was to obtain and analyze suppressor mutants that would regain the ability to import peptides despite the absence of Ubr1 [50].
By 1998, this approach identified Cup9, a previously known [279, 280] homeodomain protein that played a (mechanistically undefined) role in copper homeostasis. Byrd and Turner found that Cup9 was a transcriptional repressor of the Ptr2 peptide transporter and of several other genes as well [50]. (The transcriptional regulon of Cup9, which remains to be mapped in detail, contains between ~10 and ~50 genes.) They have also found that the Cup9 repressor of PTR2 is a short‑lived protein (t 1/2 of ~5 min) whose degradation requires Ubr1. This explained the necessity of the Arg/N-degron pathway (Fig. 3G), which destroys Cup9, for making possible the expression of Ptr2.
In 1998, this discovery of the Ubr1-Cup9-Ptr2 circuit (see Fig. 5 and its legend for additional details) was the first example of a physiological process controlled by the Arg/N-degron pathway [50]. In contrast to substrates of the Arg/N-degron pathway that contained a destabilizing Nt‑residue recognizable by Ubr1 (Fig. 3G), Cup9 was targeted by Ubr1 through a non‑N‑terminal degron, as described in the next summary, below.
Discovery of the first case of autoinhibition of an E3 Ub ligase: dipeptides bearing destabilizing Nt-residues bind to Ubr1 and enable its targeting of Cup9
In 2000-2002 studies that followed the 1998 paper by Byrd and Turner [50], Turner and Fangyong Du (a graduate student in the lab) have discovered the allosteric regulation of the Ubr1 E3 by small compounds such as dipeptides bearing destabilizing Nt-residues [51, 52]. What follows is a summary of understanding attained through their work.
In the absence of extracellular di/tripeptides, the S. cerevisiae transcriptional repressor Cup9 shuts off (nearly but not completely) the expression of PTR2, which encodes the transmembrane peptide importer (see Fig. 5 and its legend for additional details). This makes yeast cells nearly (but not entirely) incapable of importing di/tripeptides. The spatially adjacent but distinct type-1 and type‑2 binding sites of the 225-kDa Ubr1 E3 Ub ligase recognize Arg/N-degrons through their binding, respectively, to basic (Arg, Lys, His) and bulky hydrophobic (Trp, Phe, Tyr, Leu, Ile) Nt-residues in either proteins or short peptides [53].
If a yeast cell finds itself in the presence of extracellular di/tripeptides, they are taken in slowly at first, because of initially low levels of the Ptr2 transporter in the plasma membrane. However, the imported di/tripeptides (a quasi-random mix of them, derived from a food source that the cell sits on or is immersed in) that bear destabilizing Nt-residues can bind to the type-1/2 sites of Ubr1. These interactions have been found to cause, allosterically, a conformational transition that "activates" (unmasks) a separate (third) binding site of Ubr1, the one that recognizes a degron of the Cup9 repressor (Fig. 5) [51, 52].
The resulting strong increase of the allosterically activated form of Ubr1 targets Cup9 for degradation much more efficaciously, since a larger fraction of Ubr1 molecules is now in the activated form [51, 52]. As a result, the in vivo t 1/2 of Cup9 is decreased from ~5 min in wildtype (UBR1) cells in the absence of extracellular peptides to less than 1 min in the presence of peptides. Consequently, the PTR2 gene is derepressed and the Ptr2 transporter is overproduced, greatly increasing the capacity of cells to import di/tripeptides. By contrast, in ubr1Δ cells the levels of metabolically stabilized Cup9 strongly increase and shut off the expression of PTR2, thus accounting for the inability of ubr1Δ cells to import di/tripeptides. [51, 52].
This positive-feedback circuit enables both the budding yeast S. cerevisiae and the fission yeast Schizosaccharomyces pombe to detect the presence of extracellular di/tripeptides and to react by accelerating their uptake (Fig. 5) [51, 52, 281].
Both the 2000 findings by Turner et al. [51] and our later studies (J.H. Oh., J.Y. Hyun and A.V., unpublished data) indicated that the Cup9 degron recognized by Ubr1 is a version of C-degron.
Regulation of peptide import through phosphorylation of the Ubr1 N-recognin E3
In 2008, Cheol-Sang Hwang (then a postdoc in our lab) carried out extensive mass spectrometry (MS)-based and site-directed mutagenesis-based analyses of phosphorylation of the 225-kDa S. cerevisiae Ubr1 E3 [122]. Ubr1 was found to be phosphorylated in vivo at multiple sites, including Ser-300 and Tyr-277. Hwang showed that Ser-300 was phosphorylated by the type-I casein kinases Yck1 and Yck2. It was found, then, that this phosphorylation step makes possible (‘‘primes'') the subsequent (presumably sequential) phosphorylations of Ubr1 on Ser-296, Ser‑292, Thr-288, and Tyr-277 by Mck1, a kinase of the glycogen synthase kinase 3 (Gsk3) family [122]. Phosphorylation of Ubr1 on Tyr-277 by Mck1 is a previously undescribed example of a cascade-based tyrosine phosphorylation by a Gsk3-type kinase outside of autophosphorylation. Hwang also found that the Yck1/Yck2-mediated phosphorylation of Ubr1 on Ser-300 plays a major role in the Cup9-mediated control of peptide import (see above) by the Arg/N-degron pathway. In contrast to phosphorylation on Ser-300, the subsequent (primed) phosphorylations, including the one on Tyr-277, have at most minor effects on the currently known properties of Ubr1, including the Ubr1-mediated regulation of peptide import.
Recent cryo-EM analyses of S. cerevisiae Ubr1 by the laboratory of Minglei Zhao (Pan et al. [282]) have revealed the 3-D structure of full length Ubr1 at the ~3.3 Å resolution (in a complex with an E2 Rad6-Ub thioester and a synthetic, peptide-sized N-degron) This success [282], after several previous attempts, by excellent structural groups, to determine 3-D structure of Ubr1, opens up a number of previously unavailable possibilities to address the design and functions of the Arg/N-degron pathway, including the roles of Ubr1 phosphorylation [122].
The Ac/N-degron pathway
~60% and ~80% of, respectively, S. cerevisiae and human proteins are irreversibly Nα-terminally acetylated (Nt-acetylated) by Nt-acetylases [169]. The 2010 discovery of Ac/N-degrons by Cheol-Sang Hwang and Anna Shemorry in our lab [123] identified a new role of Nt-acetylation. Until then the functional significance of this universally present modification was largely obscure. The Ac/N-degron pathway targets proteins for degradation by recognizing their Nt‑acetylated Nt-residues (Fig. 3F) [123, 132, 175, 177]. The currently known E3 Ub ligases (Ac/N-recognins) of this pathway are the ER membrane-embedded yeast Doa10 and its mammalian counterpart Teb4, and also Not4, the E3 subunit of Ccr4-Not, a multifunctional cytosolic/nuclear complex [123, 132, 177].
In 2011, the laboratory of Brenda Schulman showed that the Nt-Ac group of a subunit in a protein complex usually increases thermodynamic stability of the complex [283]. The affinity‑enhancing effect of Nt-acetylation can account for at least intermittently long half-lives of many Nt‑acetylated proteins. Specifically, natural Ac/N-degrons tend to be conditional, owing to their rapid sequestration within cognate protein complexes [177]. The functions of the Ac/N‑degron pathway (Fig. 3F) include protein quality control and the regulation of input protein stoichiometries in vivo. For example, S. cerevisiae Nt-Ac-Cog1, a physiological short‑lived Ac/N-degron substrate, can be made long-lived by coexpressing Cog2 or Cog3, the Cog1-binding subunits of the Golgi‑associated COG complex [177]. Analogously, S. pombe Nt‑Ac-Hcn1, a short-lived Ac/N-degron substrate, can be stabilized by coexpressing Cut9, a cognate ligand of Hcn1 in the APC/C Ub ligase [177].
The Pro/N-degron pathway
When glucose is low or absent, cells synthesize it through gluconeogenesis. In yeast, the main gluconeogenesis-specific cytosolic enzymes are the Fbp1 fructose-1,6-bisphosphatase, the Icl1 isocitrate lyase, the Mdh2 malate dehydrogenase, and the Pck1 phosphoenolpyruvate carboxykinase. When S. cerevisiae grows on a nonfermentable carbon source such as, e.g. ethanol, the gluconeogenic enzymes are expressed and long-lived. Transition to a medium containing glucose inhibits the synthesis of these enzymes and induces their degradation, as shown mediated by the multisubunit GID Ub ligase and the proteasome, as shown previously by Dieter Wolf and colleagues.
In 2017 Stanley (Shun-Jia) Chen, a postdoc, and his colleagues in our lab discovered that Gid4, a subunit of GID, is the N-recognin of a proteolytic system that we termed the Pro/N-degron pathway (Fig. 3E) [135]. Gid4 was shown to recognizes a substrate through its Nt-Pro residue or a Pro at position 2, in the presence of distinct (but non-unique) adjoining sequence motifs. The gluconeogenic enzymes Fbp1, Icl1, Mdh2, and Pck1 bear either Nt-Pro or a Pro at position 2, and are conditionally short‑lived substrates of the Gid4-dependent Pro/N-degron pathway (Fig. 1E) [135, 136, 172]. The 3-D structure of Gid4 comprises an antiparallel β-barrel that contains a deep and narrow substrate-binding cleft (Fig. 2A) [136, 172].
Gid10 as an alternative N-recognin of the Pro/Ndegron pathway
In 2019, while studying the S. cerevisiae Gid4 [135], Artem Melnikov and Stanley (Shun-Jia) Chen in our lab identified a similar but uncharacterized yeast protein (YGR066C), which we named Gid10 [139]. A screen for N-terminal peptide sequences that can bind to Gid10 showed that substrate specificities of Gid10 and Gid4 overlap but are not identical. Gid10 is not expressed under usual (unstressful) growth conditions, but is induced upon starvation or osmotic stresses. Using protein binding analyses and degradation assays with protein substrates of GID, we showed that Gid10 can function as a specific N-recognin of the Pro/N-degron pathway [139].
Evolution of substrates and components of the Pro/N-degron pathway
As described above, Gid4, a subunit of the ubiquitin ligase GID, is the recognition component of the Pro/N-degron pathway. Gid4 targets proteins in particular through their Nt-Pro residue. In S. cerevisiae and other Saccharomyces yeasts, the gluconeogenic enzymes Fbp1, Icl1, and Mdh2 bear Nt-Pro and are conditionally destroyed by the Pro/N-degron pathway [135, 139].
However, in mammals and in many non-Saccharomyces yeasts, for example, in Kluyveromyces lactis, these enzymes lack Nt-Pro. Stanley (Shun-Jia) Chen and Artem Melnikov in our lab used K. lactis to explore evolution of the Pro/N-degron pathway [140].
One question to be addressed was whether the presence of non-Pro Nt residues in the K. lactis Fbp1, Icl1, and Mdh2 proteins was accompanied, on evolutionary time scales (S. cerevisiae and K. lactis diverged at least 20 million years ago), by a changed specificity of the Gid4 N-recognin. Yeast-based two-hybrid binding assays and protein-degradation assays were used to show that the non-Pro (Ala) Nt residue of K. lactis Fbp1 makes this enzyme long-lived in K. lactis. It was also found that the replacement, through mutagenesis, of Nt-Ala and the next three residues of K. lactis Fbp1 with the four-residue Nt-PTLV sequence of S. cerevisiae Fbp1 sufficed to make the resulting "hybrid" Fbp1 a short-lived substrate of Gid4 in K. lactis. We consider a blend of quasi-neutral genetic drift and natural selection that can account for these and related results. To our knowledge, this work is the first study of the Ub system in K. lactis, including the development of the first protein chase-degradation assay (based on the antibiotic blasticidin) suitable for use with this organism [140].
Aminopeptidases trim Xaa-Pro proteins, initiating their degradation by the Pro/N-degron pathway
As described in the preceding summary, studies by Chen and colleagues have defined the S. cerevisiae Pro/N-degron pathway and its Pro/N-recognins such as Gid4 and the stress-inducible Gid10 [135, 139, 141].
Gid4 targets proteins through the binding to their Nt-Pro residue [135]. Gid4 is also required for degradation of Nt-Xaa-Pro (Xaa is any amino acid residue) proteins such as Nt-[Ala-Pro]-Aro10 and Nt-[Ser-Pro]-Pck1, with Pro at position 2.
In 2021, Stanley (Shun-Jia) Chen, Leehyeon Kim, Hyun Kyu Song and myself, in a collaboration between our lab and the laboratory of Hyun Kyu Song (Korea University, Seoul, South Korea) have shown that specific aminopeptidases function as components of the Pro/Ndegron pathway by removing Nt-Ala or Nt-Ser and yielding Nt-Pro, which can be recognized by the Gid4‑GID Ub ligase [284].
Nt-Ala is removed by the previously uncharacterized aminopeptidase Fra1. The activity of Fra1 is shown to be essential for the GID-dependent degradation of Nt-[Ala-Pro]-Aro10. Fra1 can also trim a protein bearing the Nt-[Ala-Pro-Pro-Pro] sequence (stopping immediately before the last Pro), and thereby can target for degradation the resulting (trimmed) protein. We also found that Nt-Ser, in the Nt-sequence Ser-Pro, is removed largely by the mitochondrial/cytosolic nuclear aminopeptidase Icp55 [284]. These advances are relevant to eukaryotes from fungi to animals and plants, since Fra1, Icp55, and the GID ubiquitin ligase are conserved in evolution. In addition to discovering the mechanism of targeting of Xaa-Pro proteins, this study has also expanded the diversity of substrates of the Pro/N-degron pathway.
The eukaryotic fMet/N-degron pathway
Nascent proteins bear Nt-Met, encoded by the AUG initiation codon. In bacteria and in eukaryotic organelles mitochondria and chloroplasts, formyltransferases Nt-formylate the Met moiety of initiator Met-tRNAs. Consequently, nascent bacterial proteins start with Nt-fMet. In contrast, proteins synthesized by the cytosolic ribosomes of eukaryotes bear unformylated Nt-Met, which is often cotranslationally Nt-acetylated, resulting in Ac/N-degrons (Fig. 1F) [123, 132, 175, 177].
In 2015, it was found that Nt-fMet residues of nascent bacterial proteins can act as bacterial N-degrons, termed fMet/N-degrons (Fig. 1C) [131]. Remarkably, it was recently discovered that Nt‑formylation of proteins, previously thought to be confined to bacteria and bacteria-derived eukaryotic organelles, can also occur at the start of translation by the cytosolic ribosomes of a eukaryote such as S. cerevisiae (Fig. 1B) [138]. Nt-formylation of yeast cytosolic proteins is mediated by the nuclear DNA-encoded Fmt1 formyltransferase, whose translocation from the cytosol to the inner matrix of mitochondria was found to be not as efficacious, even under normal conditions, as had previously been assumed, and is strongly impaired under conditions of stationary phase and other stresses [138]. The cytosolic retention of Fmt1, and the resulting upsurge in the levels of Nt-formylated cytosolic proteins in nutritionally stressed cells requires Gcn2, a protein kinase [138]. It was also discovered that Nt-formylated cytosolic proteins are targeted for selective degradation by the Psh1 E3 Ub ligase, which acts as the fMet/N-recognin of the previously unknown eukaryotic fMet/N-degron pathway (Fig. 3B).
Drug transporters, gene amplification and AT-DNA binding proteins
Briefly mentioned, below, are some studies of the 1980s, when my laboratory was at MIT, that addressed problems outside the Ub system.
MDR1, a multidrug transmembrane transporter
In 1984, Igor Roninson (then a postdoc) and I collaborated with the laboratory of David Housman at MIT to identify and clone the first mammalian gene that encoded a major multidrug transporter, termed MDR1 [285]. This transmembrane protein was the first member of a large family of transporters, some of which are induced by stress. One function of MDR-type transporters is to mediate the export of potentially or actually toxic (largely hydrophobic) compounds. Increased activity and/or abundance of multidrug transporters in cancer cells are a major cause of drug resistance in cancer therapy. By making possible both dissection of MDR1 and the cloning of other MDR-type transporters, the above 1984 breakthrough [285] gave rise to a large field in cancer research.
Induced gene amplification
Gene amplification in mammalian cells was discovered in 1978 by Frederic Alt and the late Robert Schimke. In 1981, I found that growth factors such as hormones or tumor promoters can greatly increase the frequency of gene amplification under conditions of cytotoxic stress [286]. (The lab was small then, and I could still work at the bench.) A follow-up study in 1983 with James Barsoum (then a graduate student) confirmed and extended these results [287]. Analogous gene amplification events contribute to rapid evolution of cancer cells in a tumor, and to the emergence of drug-resistant cells during anticancer therapy. Thea Tlsty and Robert Schimke independently demonstrated, also in 1981, the same phenomenon of induced (accelerated) gene amplification.
HMG-I/Y, datin and D1 as AT-DNA Binding Proteins
In the 1982 study of Drosophila nucleosomes that revealed their preferential ubiquitylation in a transcribed region such as the HSP70 gene [209], we noticed that nucleosomes containing a ~50 kDa protein called D1 [288] were entirely devoid of Ub-H2A. Another 1982 study by Louis Levinger (then a postdoc) and myself showed that the D1 protein preferentially binds to (A+T)‑rich DNA in vitro, including the 1.672 and 1.688 satellite DNA repeats, and is a component of isolated nucleosomes containing these centromeric satellite DNAs [289]. Later studies [290, 291] indicated that D1, which contains 10 copies of the "AT-hook" domain, a motif that interacts with the minor groove of AT-rich DNA, is a part of heterochromatin-associated complexes that mediate transcriptional repression.
During the early 1980s (before Ub studies "displaced" all other work in the lab), the above study of D1 increased my interest in AT-DNA binding proteins, in part because of their relevance to sequence-specific nucleosome arrangements in chromatin. Our projects in this arena led to discoveries of two new proteins. In 1984, Francois Strauss (then a postdoc) and I identified and purified a green monkey protein, eventually termed HMG-I/Y (we called it, initially, alpha‑protein), that was found to bind to three specific AT-rich sites in the nucleosome-sized DNA repeat of the monkey alpha-satellite DNA [292]. Later studies by others showed that HMG-I/Y, which contains AT-hook domains, plays a role in the assembly and function of DNA‑associated multiprotein complexes that mediate transcriptional activation [290, 291].
In 1989, Edward Winter (then a postdoc) and I searched for AT-DNA binding proteins in yeast, and identified a 248-residue protein, named datin, which specifically binds to DNA containing oligo(dA)-oligo(dT) tracts [293]. Such tracts are common in the intergenic regions of S. cerevisiae and other organisms. The 1989 discovery of datin was our lab's last contribution to chromosome research for many years to come. By that time, Ub studies supplanted all other work in the lab.
ZIERLER: Returning to the problem of sleep: what is the idea about the cause of sleep that you mentioned above?
VARSHAVSKY: Sleep is universal among mammals and other vertebrates. Animals with much smaller nervous systems, such as insects, also sleep. Maladaptive aspects of sleep include elevated dangers of predation during sleep, the neglect of territorial defense and foraging for food, and the loss of parental care and mating opportunities. It is unclear what features of sleep did not allow these detrimental consequences of sleep (its fitness costs) to cause, through natural selection, a significant shortening or elimination of sleep during evolution. In sum, the molecular‑level cause of sleep is largely unknown. There are quite a few ideas about that cause or causes. Some of these ideas are constructive and interesting, but there's little definitive understanding.
In 2012 [11] and in more details in 2019 [12], I suggested that the cause of sleep may stem from cumulative effects of numerous intracellular and extracellular protein fragments. Such fragments are continually produced, in the brain and in other organs, through nonprocessive cleavages of many proteins by a multitude of intracellular, intramembrane, and extracellular proteases. Protein fragments can be beneficial but may be toxic as well, and most of them must be selectively eliminated in postmitotic (no longer dividing) cells such as, for example, neurons or cells of the skeletal muscle. According to the proposed conjecture, termed the fragment generation (FG) hypothesis, some protein fragments may be eliminated too slowly during wakefulness. For example, selective degradation of accumulating fragments might be delayed within natural protein aggregates such as, for instance, postsynaptic densities (PSDs) in postsynaptic neurons that abut synaptic clefts in excitatory synapses [12]. Delays in selective elimination of protein fragments might be caused by an impeded diffusion of the ∼3,000-kDa 26S proteasome, a component of the Ub system and the largest intracellular protease. The 26S proteasome is about the size of the large ribosomal subunit. This proteolytic machine recognizes polyubiquitylated proteins (whose poly-Ub chains are generated by specific Ub ligases) and processively destroys ubiquitin-bearing proteins. One possibility (at present, not more than a verifiable conjecture) is that a major function of sleep involves a partial and reversible expansion of PSDs (and analogous protein meshworks) during sleep, allowing an accelerated destruction of PSD-localized fragments by the proteasome.
The FG hypothesis also posits the existence of FG sentinels, i.e., specific proteins (or their natural fragments) that signal to sleep-regulating circuits that the levels of fragments are going up [12]. Interestingly, natural fragments of specific proteins are known to be overproduced in the brain during epileptic seizures. Overproduction of specific protein fragments during a seizure is strong enough to make them readily detectable by immunoblotting. Also tellingly, reactions of people (and rodents such as mice) to seizures often include postictal (post-epileptic) sleep, in agreement with a prediction by the FG hypothesis. (This is a circumstantial evidence, not a proof.) In sum, the FG hypothesis suggests a specific, molecular, and possibly chief reason because of which sleep evolved in the first place [12].
The laboratory of Lior Appelbaum (Bar-Ilan University, Israel) has recently discovered a causal involvement of the DNA repair enzyme poly(ADP-ribose) polymerase 1 (Parp1) in processes that prolong sleep after sleep deprivation, and possibly in other aspects of sleep as well, using both zebrafish and mice [294]. Previous studies (cited in Zada et al. [294]) showed that an enriched environment during wakefulness (resulting in enhanced activities of neuronal circuits) causes upregulates DNA double-strand breaks (DSBs) in neurons. The Parp1 enzyme is a major early contributor to the detection and eventual repair of DSBs, and has now been identified as a contributor to sleep drive as well [294]. Thus, cleavages (not yet in proteins but in DNA!) are apparently important in sleep causation.
I would love to verify the FG hypothesis, and to falsify it if it's incorrect. We had problems, so far, with developing experimental tests of FG that would be rigorous (causal), as distinguished from merely circumstantial (correlational) lines of evidence.
New biochemical and genetic methods
A scientific study is constrained by the quality and sophistication of its tools. Hence my lifelong interest in developing new methods. I mentioned above 2-D electrophoretic techniques for analyzing intermediates in DNA replication that were introduced in 1980-1981, by Olof Sundin and myself (Fig. 1). These methods led to the discovery of the multicatenane pathway of chromosome cohesion/segregation [21, 22], and are still in use today.
Low ionic strength gel electrophoresis and gel shift assay
In 1975, when I still worked at IMB (Moscow, Russia), my colleagues and I introduced a method for gel electrophoresis in nondenaturing buffers of low (~10 mM) ionic strength [206, 207]. In such buffers, decreased levels of counterions increased both stability and mutual repulsion of, for example, negatively charged nucleoprotein complexes, and thereby greatly reduced their aggregation during gel electrophoresis. In a 1976 application of this technique, the forerunner of the gel shift assay, we employed it to fractionate isolated mononucleosomes and oligonucleosomes at a previously unattainable resolution [207].
In 1981, the laboratories of Donald Crothers [295] and Arnold Revzin [296] independently employed a similar nondenaturing gel electrophoresis, termed later the gel shift assay [297], to detect and measure the interactions of specific DNA-binding proteins (e.g., the Lac repressor) with their cognate DNA operators.
In 1984, Francois Strauss (then a postdoc) and I demonstrated that the gel shift assay, until then used exclusively with DNA and purified proteins, can detect specific DNA-binding proteins in the presence of nonspecifically binding ones, provided that a sample contains a sufficient excess of "nonspecific" DNA [292]. This simple modification of gel shift assays made possible the detection of sequence-specific DNA-binding proteins in crude cell extracts. Since then, the resulting assay has become a major tool in studies of gene expression.
The chromatin immunoprecipitation (ChIP) assay
In 1988, Mark Solomon (then a graduate student), Pamela Larsen (then a postdoc) and I developed the chromatin immunoprecipitation (ChIP) assay (it was termed ChIP by later users of this method) [298]. In this technique, intact cells are covalently and extensively crosslinked with a reagent such as formaldehyde (HCHO), followed by a hydrodynamic disruption of fixed cells that includes extensive fragmentation of their (HCHO-crosslinked) chromosomes, and immunoprecipitation of resulting (HCHO-crosslinked) chromatin fragments with an antibody to a protein of interest. Subsequent thermal reversal of crosslinks makes possible the identification (through hybridization or DNA sequencing) of specific genomic sites that are bound, in vivo, to a protein of interest under different physiological conditions [298].
Over the years, several improvements, by other groups, of the original ChIP assay (including DNA sequencing instead of DNA hybridization) have made this method a particular versatile tool in chromosome research. The earlier use of HCHO-mediated crosslinking, both in our 1978‑1979 discovery of the exposed (nucleosome-depleted) region in the SV40 minichromosomes [19, 20] and in developing the 1988 ChIP assay [298], stemmed from my earlier use of HCHO as a crosslinking tool during chromosome studies of the 1970s, in Moscow, Russia [299].
Hypersensitivity to heavy water, a generally applicable conditional phenotype
One of our lab's last "non-Ub" contributions in the 1980s was the invention, by Bonnie Bartel (then a graduate student) and myself, of a new way to make conditional mutants [300]. Although temperature-sensitive (ts) mutants were a mainstay of genetic analysis for decades, my first encounters with them, in the early 1980s, suggested that this approach could stand an improvement. One problem is the leakiness of ts mutants, i.e., a nonzero activity of a ts protein at nonpermissive temperature. In addition, producing a ts version of a protein of interest is often an iffy affair that requires extensive mutagenesis. Might there be other, also generally applicable, types of conditional mutants? Might there be a perturbant as pervasive as temperature? One day in 1986, I realized that the answer may be yes: heavy water (D2O)!
Chemically, D2O is indistinguishable from H2O in all respects except for quantitative shifts in, for example, conformational stability of folded proteins and nucleic acids. The idea was simplicity itself: determine a maximal concentration of D2O that is still compatible with a near‑normal growth of an organism of interest, such as S. cerevisiae. Thereafter quasi-randomly mutagenize the cells and carry out a screen for mutants that are hypersensitive to D2O, i.e., for mutants (we called them ds, D2O-sensitive) that cannot grow at a concentration of D2O that is still nontoxic to wild-type cells. If such mutants could be obtained, straightforward assays could be used, then, to determine whether most of the organism's essential genes can be mutated to a conditional ds phenotype.
I did preliminary experiments, which suggested that the ds technique may be feasible. Bonnie Bartel got interested in this approach, and soon demonstrated that yeast ds mutants could be readily produced. Moreover, apparently any or almost any gene could be mutated to a ds allele. Interestingly, we found that most ds mutants were not ts, and vice versa. In other words, the ds and ts techniques tapped largely different sets of mutations in cellular genes. Although the ds method [300] is technically straightforward and possibly superior to ts in some settings, the ts technique continues to be the predominant approach, in part because of its comparative ease and familiarity. Described below is yet another method for constructing conditional mutants, developed by us in 1994 [301].
The ubiquitin fusion technique
In 1986, Bachmair, Finley and I invented the Ub fusion technique, which led to the discovery of the first degrons and the Arg/N-degron pathway (called, then, the "N-end rule pathway") [58]. DNA-encoded linear Ub fusions to other proteins mimic natural Ub fusions that are encoded by Ub genes [67, 71]. We found that linear Ub fusions are cotranslationally cleaved at the Ub‑protein junction by DUBs irrespective of the identity of a residue at the junction's Ct-side, with proline (Pro) being a single (and partial) exception [58, 59]. Even after more than three decades, the resulting Ub-fusion technique, in which site-directed mutagenesis is used to alter the identity of a residue at the Ub-protein junction, is still the method of choice for placing, in vivo, a desired residue at the N-terminus of a protein of interest [58, 302]. The requirement for a special "technique" to alter the N-termini of proteins stems from constraints of the genetic code, as all nascent proteins start with the Met residue. Met-aminopeptidases (MetAPs) would cleave off the N-terminal Met if only if a residue at position 2, to be made N-terminal after cleavage, has a small enough side chain [302]. Hence the necessity of a "bypass" method to alter Nt‑residues at will in vivo [58]. Several descendants of the Ub fusion technique that were developed in the lab over the last three decades are briefly described below.
The ubiquitin reference technique
This 1996 method, by Frédéric Lévy (then a postdoc) and other colleagues in our lab, uses Ub fusion constructs encoding both a protein of interest and a "reference" protein, with two proteins cotranslationally coexpressed at the (initially) equimolar levels [302, 303]. Through a "built-in" reference protein, this method increases the accuracy of pulse-chase assays [51, 115].
Heat-activated degron (ts-degron)
This 1994 method, by Jürgen Dohmen, Pei Pei Wu (then postdocs in our lab) and myself, allows one to produce ts alleles of specific proteins without the (usual) necessity of random mutagenesis and without altering a protein's amino acid sequence [301]. The ts-degron method involves a portable N-degron that can be activated by an increased temperature. A protein of interest is linked to such a degron, and becomes short lived in vivo at that (increased) temperature [301, 304].
The ubiquitin translocation technique
This 1994 method, by Nils Johnsson (then a postdoc) and myself, allows one to probe, in vivo, kinetic aspects of protein translocation across membranes [305]. A fusion in which the Ub moiety is placed between a signal sequence and a reporter domain is cleaved by DUB enzymes in the cytosol unless the fusion can "escape" into a compartment such as the endoplasmic reticulum (ER). The critical step involves a rapid folding of the newly formed Ub moiety, which precludes its translocation and makes possible the cleavage by DUBs after its last residue. However, if a sufficiently long spacer is present between the signal sequence and Ub, then by the time the Ub polypeptide emerges from the ribosome and is ready to fold, the ribosome is already docked at the transmembrane channel, allowing the translocation of both the Ub moiety and the downstream reporter domains of a fusion into the ER. These mutually exclusive outcomes make it possible to probe, by varying the length of spacer and other parameters, both kinetic and stochastic aspects of targeting in protein translocation, and to compare the strengths of different signal sequences in vivo. The Ub translocation technique [305] is also applicable to non-ER translocation.
Split-ubiquitin assay for in vivo protein interactions
This 1994 method, also by Nils Johnsson and myself [2], makes possible both the detection of a protein-protein interaction and its monitoring as a function of time, at the natural sites of this interaction in living cells. The latter feature of the split-Ub technique is one of its differences vis‑à-vis methods such as the classical two-hybrid assay, where interacting protein domains must "make" it to the nucleus and transcriptional promoter settings. When a ~40-residue Ct-half of the 76-residue Ub (Cub) was expressed as a fusion to a reporter protein, the fusion was cleaved only if the Nt-half of Ub (Nub) was also expressed in the same cell. Thus the two halves of a single‑domain protein could coalesce in vivo to produce a quasi-native monomeric Ub moiety, thereby allowing the cleavage by DUBs at the Ub-reporter junction. This reconstitution of quasi‑native Ub from its fragments was not observed with a specific missense mutant of the Nub half of Ub. However, if Cub and the altered Nub were each linked to polypeptides that physically interacted in vivo, the cleavage of the reporter fusion containing Cub was restored. This approach yielded an assay for in vivo protein interactions [2]. Variants of the split-Ub assay were developed after 1994 and employed to dissect specific protein interactions in vivo, or to screen for new interactions [3, 306-308]). The invention of the split-Ub technique [2] gave rise to the field of monomeric protein fragment complementation assays (PCAs) and a number of useful designs that include split dihydrofolate reductase (DHFR) [309], split green fluorescent protein (GFP) [310], and other split proteins.
The ubiquitin sandwich technique
"Cotranslational" degradation refers to a processive destruction of a polypeptide in vivo while it is still a part of a ribosome-associated peptidyl-tRNA. How to detect and measure such degradation? In the 2000 Ub sandwich technique by Glenn Turner (then a graduate student) and myself [116], the polypeptide to be examined for cotranslational degradation, termed B, is sandwiched between two reporter domains, A and C. The A, B and C domains are connected by Ub moieties, yielding a linear AUb-BUb-CUb fusion. DUB enzymes cotranslationally cleave this fusion, generating AUb, BUb, and CUb. After the initial cleavage at the AUb-BUb junction, the activity of DUBs results in a kinetic competition between two mutually exclusive fates during the synthesis of AUb-BUb-CUb: cotranslational cleavage at the BUb-CUb junction, which produces the long-lived CUb module, or, alternatively, the cotranslational, processive degradation of the entire BUb-CUb nascent chain by the proteasome that destroys the Ub moiety between B and C before it can be recognized by DUBs. The resulting decrease in CUb relative to AUb reflects both the occurence and extent of cotranslational degradation of domain B [116]. Using this method, we found that more than 50% of nascent protein molecules bearing an N‑degron can be degraded cotranslationally, never reaching their mature size before their destruction by processive proteolysis [116]. Thus, the folding of nascent proteins, including abnormal ones, may be in kinetic competition with pathways that target these proteins for the cotranslational degradation.
The subunit decoy technique
This generally applicable method for analyzing the in vivo regulation of subunit stoichiometries in oligomeric proteins was developed in 2013 as a part of the study, by Anna Shemorry (then a graduate student) and Cheol-Sang Hwang (then a postdoc) in our lab, that aimed to analyze the Ac/N‑degron pathway of protein degradation [177].
Some results of that study [177] suggested that the observed metabolic stabilization of AcMD‑Cog1 (Ac-Met-Asp-Cog1, a natural Ac/N-degron substrate in S. cerevisiae) stemmed from sequestration of the Ac/N-degron AcMD‑Cog1 by other subunits of the COG complex during the assembly of that (degradation-resistant) complex.
This possibility was addressed using a a generally applicable approach referred to as the subunit decoy technique [177]. First, a C-terminally epitope-tagged protein of interest is expressed at its endogenous level from its native chromosomal location and transcriptional promoter. Second, the same protein but C-terminally tagged with a different epitope is also expressed in these cells. Cells expressing wildtype levels of AcMD-Cog113myc (Ct-tagged with 13 myc epitopes) from the chromosomal COG1 locus were transformed either with a vector alone or with a plasmid expressing the otherwise identical AcMD-Cog13f (C-terminally tagged with three flag epitopes) from the constitutive PADH1 promoter. Whereas the endogenous AcMD-Cog113myc was long-lived in the absence of ectopically expressed AcMD-Cog13f , the same endogenous AcMD-Cog113myc became a short-lived protein in the presence of the (also short-lived) AcMD-Cog13f [177]. In another design of the subunit decoy experiment, cells expressing wildtype levels of AcMD‑Cog13ha (C-terminally tagged with three ha epitopes) from the chromosomal COG1 locus were transformed with a plasmid expressing the otherwise identical AcMD-Cog13f from the galactose-inducible PGAL1 promoter. In glucose medium, where AcMD-Cog13f was not expressed, the endogenous AcMD-Cog13ha was long-lived. In striking contrast, the same endogenous AcMD-Cog13ha became short-lived in the presence of galactose, which induced the expression of AcMD-Cog13f [177].
In the subunit decoy method, a C-terminally epitope-tagged protein is expressed at its endogenous (wildtype) level in the absence or presence of the same protein that is alternatively tagged and overexpressed. As described for the AcMD-Cog1 protein above, one result of this generally applicable strategy is the displacement of the bulk of the previously protected protein subunit of a complex, making that subunit vulnerable to attack by a cognate proteolytic pathway (in the case above, the Ac/N-degron pathway) [177].
A reference-based protein degradation assay without global translation inhibitors
Although it is widely appreciated that the use of global translation inhibitors, such as cycloheximide, in protein degradation assays may result in artifacts, these inhibitors continue to be employed, owing to the absence of robust alternatives. Jang-Hyun Oh and Stanley (Shun-Jia) Chen (postdocs in our lab) developed a protein degradation assay, termed the promoter reference technique (PRT), which has two advantageous features: a reference protein and a gene-specific inhibition of translation [180].
In PRT assays, one measures, during a chase, the ratio of a test protein to a long-lived reference protein, a dihydrofolate reductase (DHFR). The test protein and DHFR are coexpressed, in the S. cerevisiae, on a low-copy plasmid from two identical PTDH3 promoters containing additional, previously designed (by Suess and colleagues [311]) DNA elements. Once transcribed, these elements form 5'-RNA aptamers that bind to the added tetracycline, which represses translation of aptamer-containing mRNAs. The selectivity of repression avoids a global inhibition of translation [180].
This selectivity is particularly important if a component of a relevant proteolytic pathway (e.g. a specific Ub ligase) is itself short-lived. In the cited paper [180], PRT was used in the context of the Pro/N-degron pathway, whose substrates include the short-lived Mdh2 malate dehydrogenase. Mdh2 is targeted for degradation by the Gid4 subunit of the GID ubiquitin ligase. Gid4 is also a metabolically unstable protein. Through analyses of short-lived Mdh2 as a target of short-lived Gid4, the cited paper [180] illustrated the advantages of PRT over degradation assays that lack a reference and/or involve a global translation inhibitor. By now, PRT-based degradation assays have also been used in settings outside the Pro/N-degron pathway (e.g., [137]).
ZIERLER: Alex, it has been wonderful spending this time with you. On what point shall we conclude?
VARSHAVSKY: There was a good writer in Russia, named Yury Trifonov (1925‑1981). An episode from a novel by him: the narrator sits on a bench in a park and reads a book. An old woman walks by and takes a seat on the same bench. After a while, the woman says, to nobody in particular: "The only meaningful thing is to love and be loved. Everything else has no meaning whatsoever." The narrator is startled, then goes back to reading.
I totally agree with the woman on the bench. An addendum, though. There's also a love for a nonliving entity, such as science. The illusion of meaning that this attraction engenders can be amazingly strong. The woman on the bench might not have known about that love. I do, first‑hand.
A scientist's life is largely incidental, tangential to one's discoveries and inventions, in a profession that tends to swallow a person whole. Our propensity to be curious about other people obscures the fact that it's essentially immaterial that Isaac Newton was not a kind man, or that Johannes Kepler had to defend his mother in court against the charge of being a witch (she was acquitted). To think of Newton or Kepler is to think of their work. The same applies to all of us in this remarkable guild, irrespective of the scale of one's accomplishment.
2022
Brief CV
Name: Alexander J. Varshavsky
Date and Place of Birth: November 8, 1946, Moscow, Russia
Citizenship: U.S. citizen
Address: Division of Biology and Biological Engineering, 147-75
California Institute of Technology
1200 E. California Blvd., Pasadena, CA 91125, USA
Telephone: 626-395-3785 (office phone); 818-606-1908 (cell phone)
Email: [email protected]
Web: http://www.bbe.caltech.edu/people/alexander-j-varshavsky
Academic Appointments and Education:
1970: B.S. in Chemistry, Moscow University, Moscow, Russia.
1973: Ph.D. in Biochemistry, Institute of Molecular Biology, Moscow.
1973-1976: Research Fellow, Institute of Molecular Biology, Moscow.
1977-1980: Assistant Professor, Dept. of Biology, M. I. T., Cambridge, MA.
1980-1986: Associate Professor, Department of Biology, M. I. T.
1986-1992: Professor of Biology, Department of Biology, M. I. T.
1992-2017: Howard and Gwen Lawry Smits Professor of Cell Biology, Division of Biology, California Institute of Technology, Pasadena, CA.
2017-present: Thomas Hunt Morgan Professor of Biology, Division of Biology and Biological Engineering, California Institute of Technology, Pasadena, CA.
Other Appointments:
Member, Molecular Cytology Study Section, NIH, 1983-1987.
Co-organizer, Banbury Conf. on the Ubiquitin System, Cold Spring Harbor Laboratory, 1993.
Visiting Fellow, International Institute for Advanced Studies, Kyoto, Japan, 2001.
Member, Advisory Board, Encyclopedia of Mol. Cell Biology and Mol. Medicine, 2002-2005.
Member, Advisory Board, Gairdner Foundation, Canada, 2002-2006.
Member, Advisory Board, Massry Foundation, 2002-2005.
Member, Advisory Board, Pasarow Foundation, 2002-2012.
Member, O'Connor Advisory Committee, March of Dimes Foundation, 2007-2012.
Member, Breakthrough Prize Committee, 2014-present.
Honorary Memberships:
Fellow, American Academy of Arts and Sciences, 1987.
Member, U.S. National Academy of Sciences, 1995.
Fellow, American Academy of Microbiology, 2000.
Foreign Associate, European Molecular Biology Organization, 2001.
Member, American Philosophical Society, 2001.
Fellow, American Association for Advancement of Science, 2002.
Foreign Member, European Academy of Sciences (Academia Europaea), 2005.
Awards:
Merit Award, National Institutes of Health, 1998.
Novartis-Drew Award in Biomedical Science, Novartis, Inc. and Drew University, 1998.
Gairdner International Award, Gairdner Foundation, Canada, 1999.
(with A. Hershko)
Sloan Prize, General Motors Cancer Research Foundation, 2000.
(with A. Hershko)
Lasker Award in Basic Medical Research, Albert and Mary Lasker Foundation, 2000.
(with A. Hershko and A. Ciechanover)
Shubitz Prize in Cancer Research, University of Chicago, 2000.
Hoppe-Seyler Award, Society for Biochemistry and Molecular Biology, Germany, 2000.
Pasarow Award in Cancer Research, Pasarow Foundation, 2001.
Max Planck Award, Germany, 2001.
Merck Award, American Society for Biochemistry and Molecular Biology, 2001.
(with A. Hershko)
Wolf Prize in Medicine, Wolf Foundation, Israel, 2001.
(with A. Hershko)
Massry Prize, Massry Foundation, 2001.
(with A. Hershko)
Horwitz Prize, Columbia University, 2001.
(with A. Hershko)
Wilson Medal, American Society for Cell Biology, 2002.
(with A. Hershko)
Stein and Moore Award, Protein Society, 2005.
(with A. Hershko)
March of Dimes Prize in Developmental Biology, March of Dimes Foundation, 2006.
Griffuel Prize in Cancer Research, Association for Cancer Research, France, 2006.
Gagna and Van Heck Prize, National Foundation for Scientific Research, Belgium, 2006.
Weinstein Distinguished Award, American Association for Cancer Research, 2007.
Schleiden Medal, German Academy of Sciences (Leopoldina), 2007.
Gotham Prize in Cancer Research, Gotham Foundation, 2008.
Vilcek Prize in Biomedical Research, Vilcek Foundation, 2010.
BBVA Foundation Award in Biomedicine, BBVA Foundation, Spain, 2011.
Otto Warburg Prize, Society for Biochemistry and Molecular Biology, Germany, 2012.
King Faisal International Prize in Science, King Faisal Foundation, Saudi Arabia, 2012.
Breakthrough Prize in Life Sciences, Breakthrough Foundation, 2014.
Albany Prize in Medicine and Biomedical Research, Albany Medical Center, Albany, NY, 2014.
Grande Médaille, French Academy of Sciences, 2016.
Wieland Prize, Boehringer Ingelheim Foundation, Germany, 2017.
IUBMB Medal, International Union of Biochemistry and Molecular Biology, 2019.
Total publications: 235.
Cited publications
- Vidal, M. and S. Fields (2014) The yeast two-hybrid assay: still finding connections after 25 years. Nat. Methods 11, 1203-1206.
- Johnsson, N. and A. Varshavsky (1994) Split ubiquitin as a sensor of protein interactions in vivo. Proc. Natl. Acad. Sci. USA 91, 10340-10344.
- Dunkler, A., J. Muller, and N. Johnsson (2012) Detecting protein-protein interactions with the split-ubiquitin sensor. Methods Mol. Biol. 786, 115-130.
- Varshavsky, A., Interview to Prof. I. Hargittai. , in Candid Science, I. Hargittai and M. Hargittai, Editors. 2006, Imperial College Press, London, U.K. p. pp. 311-359.
- Hoffman, R. M., Escape. 2008: Arbor Books, Inc.
- Zhu, H., H. Zhang, Y. Xu, S. Laššáková, M. Korabečná, and P. Neužil (2020) PCR: past, present and future. Biotechniques 69, 317-325.
- Knott, G. J. and J. A. Doudna (2018) CRISPR-Cas guides the future of genetic engineering. Science 361, 866-869.
- Varshavsky, A. (1998) Codominant interference, antieffectors, and multitarget drugs. Proc. Natl. Acad. Sci. USA 95, 2094-2099.
- Varshavsky, A. (1995) Codominance and toxins: a path to drugs of nearly unlimited selectivity. Proc. Natl. Acad. Sci. USA 92, 3663-3667.
- Varshavsky, A. (2007) Targeting the absence: homozygous DNA deletions as an immutable marker for cancer therapy. Proc. Natl. Acad. Sci. USA 104, 14935-14940.
- Varshavsky, A. (2012) Augmented generation of protein fragments during wakefulness as the molecular cause of sleep: a hypothesis. Prot. Sci. 21, 1634-1661.
- Varshavsky, A. (2019) On the cause of sleep: protein fragments, the concept of sentinels, and links to epilepsy. Proc Natl Acad Sci U S A 116, 10773-10782.
- Varshavsky, A., The World as Physics, Mathematics and Nothing Else, in Physical Biology: From Atoms to Medicine, A.H. Zewail, Editor. 2008, Imperial College Press, London, U.K. p. 7-22.
- Feynman, R. P., R. B. Leighton, and M. Sands, The Feynman Lectures on Physics. . Vol. 1. 1963, Reading, MA.: Addison-Wesley Publ. Co.
- Stein, H. "If your horse dies, we suggest you dismount". A bon mot attributed to American economist Herbert Stein (1916-1999).
- Varshavsky, A. (1968) Regulation of synthesis of genetic repressors in bacteria. Mol. Biol. (Russia) 2, 13-20.
- Kornberg, R. D. (1974) Chromatin structure:: a repeating unit of histones and DNA. Science 184, 868-871.
- Bakayev, V. V., T. G. Bakayeva, and A. J. Varshavsky (1977) Nucleosomes and subnucleosomes: heterogeneity and composition. Cell 11, 619-629.
- Varshavsky, A., O. Sundin, and M. Bohn (1978) SV40 minichromosome: preferential exposure of the origin of replication. Nucl. Acids Res. 5, 3469-3478.
20. Varshavsky, A., O. Sundin, and M. Bohn (1979) A 400 bp stretch of SV40 viral DNA that includes the origin of replication is exposed in SV40 minichromosomes. Cell 16, 453-466.
21. Sundin, O. and A. Varshavsky (1980) Terminal stages of SV40 DNA replication proceed via multiply intertwined catenated dimers. Cell 21, 103-114.
22. Sundin, O. and A. Varshavsky (1981) Arrest of segregation leads to accumulation of highly intertwined catenated dimers: dissection of the final stages of SV40 DNA replication. Cell 25, 659-669.
23. Holm, C., T. Goto, J. C. Wang, and D. Botstein (1985) DNA toposomerase II is required at the time of mitosis in yeast. Cell 41,
24. Uemura, T., H. Ohkura, Y. Adachi, K. Morini, K. Shiozaki, and M. Yanagida (1987) DNA topoisomerase II is required for condensation and separation of mitotic chromosomes in S. pombe. Cell 50, 917-925.
25. Dinardo, S., R. L. Voelkel, and R. L. Sternglanz (1984) DNA topoisomerase II mutant of Saccharomyces cerevisiae: Topoisomerase II is required for segregation of daughter molecules at the termination of DNA replication. Proc. Natl. Acad. Sci. USA 81, 2616-2620.
26. Steck, T. R. and K. Drlica (1984) Bacterial chromosome segregation: evidence for DNA gyrase involvement in decatenation. Cell 36, 1081-1088.
27. Shamu, C. E. and A. Murray (1992) Sister chromatid separation in frog egg extracts requires DNA topoisomerase II activity during anaphase. J. Cell Biol. 117, 921-934.
28. Skoufias, D. A., F. B. Lacroix, P. R. Andreassen, L. Wilson, and R. L. Margolis (2004) Inhibition of DNA decatenation, but not DNA damage, arrests cells at metaphase. . Mol. Cell 15, 977-990.
29. Damelin, M. and T. H. Bestor (2007) The decatenation checkpoint. Brit. J. Cancer 96, 201-205.
30. D'Ambrosio, C., G. Kelly, K. Shirahige, and F. Uhlmann (2008) Condensin-dependent rDNA decatenation introduces a temporal pattern to chromosome segregation. Curr. Biol. 18, 1084-1089.
31. Wang, L. H.-C., T. Schwarzbraun, M. R. Speicher, and E. A. Nigg (2008) Persistence of DNA threads in human anaphase cells suggests late completion of sister chromatid decatenation. Chromosoma 117, 123-135.
32. Huang, C. E., M. Milutinovich, and D. Koshland (2005) Rings, bracelet or snaps: fashionable alternatives for Smc complexes. Phil. Trans. R. Soc. B 360, 537-542.
33. Nasmyth, K. and C. H. Haering (2005) The structure and function of SMC and kleisin complexes. Annu. Rev. Biochem. 74, 595-648.
34. Milutinovich, M., E. Ünal, C. Ward, R. V. Skibbens, and D. Koshland (2007) A multi-step pathway for the establishment of sister chromatid cohesion. PLoS Genet. 3, e12.
35. Shintomi, K. and T. Hirano (2007) How are cohesin rings opened and closed? Trends Biochem. Sci. 32, 154-157.
36. Haering, C. H., A.-M. Farcas, P. Arumugam, J. Metson, and K. Nasmyth (2008) The cohesin ring concatenates sister DNA molecules. Nature 454, 297-301.
37. Chu, L., Z. Zhang, M. Mukhina, D. Zickler, and N. Kleckner (2022) Sister chromatids separate during anaphase in a three-stage program as directed by interaxis bridges. Proc. Natl. Acad. Sci. USA 119, e2123363119.
38. Yatskevich, S., J. Rhodes, and K. Nasmyth (2019) Organization of chromosomal DNA by SMC complexes. Annu. Rev. Genet. 53, 445-482.
39. Farcas, A. M., P. Uluocak, W. Helmhart, and K. Nasmyth (2011) Cohesin's concatenation of sister DNAs maintains their intertwining. Mol. Cell 44, 97-107.
40. Broderick, R. and W. Niedzwiedz (2015) Sister chromatid decatenation: bridging the gaps in our knowledge. Cell Cycle 14, 3040-3044.
41. Uhlmann, F., F. Lottspeich, and K. Nasmyth (1999) Sister-chromatid separation at anaphase onset is promoted by cleavage of the cohesin subunit Scc1. Nature 400, 37-42.
42. Varshavsky, A. (2011) The N-end rule pathway and regulation by proteolysis. Prot. Sci. 20, 1298-1345.
43. Varshavsky, A. (2019) N-degron and C-degron pathways of protein degradation. Proc. Natl. Acad. Sci. USA 116, 358-366.
44. Rao, H., F. Uhlmann, K. Nasmyth, and A. Varshavsky (2001) Degradation of a cohesin subunit by the N-end rule pathway is essential for chromosome stability. Nature 410, 955-960.
45. Hershko, A., A. Ciechanover, and A. Varshavsky (2000) The ubiquitin system. Nat. Med. 10, 1073-1081.
46. Varshavsky, A. (2006) The early history of the ubiquitin field. Prot. Sci. 15, 647-654.
47. Varshavsky, A. (2008) Discovery of cellular regulation by protein degradation. J. Biol. Chem. 283, 34469-34489.
48. Varshavsky, A. (2014) Discovery of the biology of the ubiquitin system. J. Am. Med. Assoc. 311, 1969-1970.
49. Alagramam, K., F. Naider, and J. M. Becker (1995) A recognition component of the ubiquitin system is required for peptide transport in Saccharomyces cerevisiae. Mol. Microbiol. 15, 225-234.
50. Byrd, C., G. C. Turner, and A. Varshavsky (1998) The N-end rule pathway controls the import of peptides through degradation of a transcriptional repressor. EMBO J. 17, 269-277.
51. Turner, G. C., F. Du, and A. Varshavsky (2000) Peptides accelerate their uptake by activating a ubiquitin-dependent proteolytic pathway. Nature 405, 579-583.
52. Du, F., F. Navarro-Garcia, Z. Xia, T. Tasaki, and A. Varshavsky (2002) Pairs of dipeptides synergistically activate the binding of substrate by ubiquitin ligase through dissociation of its autoinhibitory domain. Proc. Natl. Acad. Sci. USA 99, 14110-14115.
53. Xia, Z., G. C. Turner, C. S. Hwang, C. Byrd, and A. Varshavsky (2008) Amino acids induce peptide uptake via accelerated degradation of CUP9, the transcriptional repressor of the PTR2 peptide transporter. J. Biol. Chem. 283, 28958-28968.
54. Ciechanover, A., Y. Hod, and A. Hershko (1978) A heat-stable polypeptide component of an ATP-dependent proteolytic system from reticulocytes. Biochem. Biophys. Res. Comm. 81, 1100-1105.
55. Hershko, A., A. Ciechanover, H. Heller, A. L. Haas, and I. A. Rose (1980) Proposed role of ATP in protein breakdown: conjugation of protein with multiple chains of the polypeptide of ATP-dependent proteolysis. Proc. Natl. Acad. Sci. USA 77, 1783-1786.
56. Sakata, E., M. R. Eisele, and W. Baumeister (2021) Molecular and cellular dynamics of the 26S proteasome. Biochim. Biophys. Acta 1869, 140583.
57. Bard, J. A. M., E. A. Goodall, E. R. Greene, E. Jonsson, K. C. Dong, and A. Martin (2018) Structure and function of the 26S proteasome. Annu. Rev. Biochem. 87, 697-724.
58. Bachmair, A., D. Finley, and A. Varshavsky (1986) In vivo half-life of a protein is a function of its N-terminal residue. Science 234, 179-186.
59. Bachmair, A. and A. Varshavsky (1989) The degradation signal in a short-lived protein. Cell 56, 1019-1032.
60. Varshavsky, A. (1991) Naming a targeting signal. Cell 64, 13-15.
61. Varshavsky, A. (1992) The N-end rule. Cell 69, 725-735.
62. Varshavsky, A. (1996) The N-end rule: functions, mysteries, uses. Proc. Natl. Acad. Sci. USA 93, 12142-12149.
63. Finley, D., A. Ciechanover, and A. Varshavsky (1984) Thermolability of ubiquitin-activating enzyme from the mammalian cell cycle mutant ts85. Cell 37, 43-55.
64. Ciechanover, A., D. Finley, and A. Varshavsky (1984) Ubiquitin dependence of selective protein degradation demonstrated in the mammalian cell cycle mutant ts85. Cell 37, 57-66.
65. Jentsch, S., J. P. McGrath, and A. Varshavsky (1987) The yeast DNA repair gene RAD6 encodes a ubiquitin-conjugating enzyme. Nature 329, 131-134.
66. Goebl, M. G., J. Yochem, S. Jentsch, J. P. McGrath, A. Varshavsky, and B. Byers (1988) The yeast cell cycle gene CDC34 encodes a ubiquitin-conjugating enzyme. Science 241, 1331-1335.
67. Finley, D., E. Özkaynak, and A. Varshavsky (1987) The yeast polyubiquitin gene is essential for resistance to high temperatures, starvation, and other stresses. Cell 48, 1035-1046.
68. Finley, D., B. Bartel, and A. Varshavsky (1989) The tails of ubiquitin precursors are ribosomal proteins whose fusion to ubiquitin facilitates ribosome biogenesis. Nature 338, 394-401.
69. Hochstrasser, M. and A. Varshavsky (1990) In vivo degradation of a transcriptional regulator: the yeast MATalpha2 repressor. Cell 61, 697-708.
70. Özkaynak, E., D. Finley, and A. Varshavsky (1984) The yeast ubiquitin gene: head-to-tail repeats encoding a polyubiquitin precursor protein. Nature 312, 663-666.
71. Özkaynak, E., D. Finley, M. J. Solomon, and A. Varshavsky (1987) The yeast ubiquitin genes: a family of natural gene fusions. EMBO. J. 6, 1429-1439.
72. Tobias, J. W. and A. Varshavsky (1991) Cloning and functional analysis of the ubiquitin-specific protease gene UBP1 of Saccharomyces cerevisiae. J. Biol. Chem. 266, 12021-12028.
73. Baker, R. T., J. W. Tobias, and A. Varshavsky (1992) Ubiquitin-specific proteases of Saccharomyces cerevisiae. Cloning of UBP2 and UBP3, and functional analysis of the UBP gene family. J. Biol. Chem. 267, 23364-23375.
74. Bartel, B., I. Wünning, and A. Varshavsky (1990) The recognition component of the N-end rule pathway. EMBO J. 9, 3179-3189.
75. Kwon, Y. T., Y. Reiss, V. A. Fried, A. Hershko, J. K. Yoon, D. K. Gonda, P. Sangan, N. G. Copeland, N. A. Jenkins, and A. Varshavsky (1998) The mouse and human genes encoding the recognition component of the N-end rule pathway. Proc. Natl. Acad. Sci. USA 95, 7898-7903.
76. Zheng, N. and N. Shabek (2017) Ubiquitin ligases: structure, function, and regulation. Annu. Rev. Biochem. 86, 129-157.
77. Chau, V., J. W. Tobias, A. Bachmair, D. Marriott, D. J. Ecker, D. K. Gonda, and A. Varshavsky (1989) A multiubiquitin chain is confined to specific lysine in a targeted short-lived protein. Science 243, 1576-1583.
78. Johnson, E. S., D. K. Gonda, and A. Varshavsky (1990) Cis-trans recognition and subunit-specific degradation of short-lived proteins. Nature 346, 287-291.
79. Liu, F. and K. J. Walters (2010) Multitasking with ubiquitin through multivalent interactions. Trends Biochem. Sci. 35, 352-360.
80. Gallastegui, N. and M. Groll (2010) The 26S proteasome: assembly and function of a destructive machine. Trends Biochem. Sci. 35, 634-642.
81. Hampton, R. Y. and R. M. Garza (2009) Protein quality control as a strategy for cellular regulation: lessons from ubiquitin-mediated regulation of the sterol pathway. Chem. Rev. 109, 1561-1574.
82. Grabbe, C. and I. Dikic (2009) Functional roles of ubiquitin-like domain (ULD) and ubiquitin-binding domain (UBD) containing proteins. Chem. Rev. 109, 1481-1494.
83. Daulni, A. and W. P. Tansey (2009) Damage control: DNA repair, transcription, and the ubiquitin-proteasome system. DNA Repair 8, 444-448.
84. Deshaies, R. J. and C. A. P. Joazeiro (2009) RING domain E3 ubiquitin ligases. Annu. Rev. Biochem. 78, 399-434.
85. Marques, A. J., R. Palanimurugan, A. C. Matias, P. C. Ramos, and R. J. Dohmen (2009) Catalytic mechanism and assembly of the proteasome. Chem. Rev. 109, 1509-1536.
86. Ravid, T. and M. Hochstrasser (2008) Diversity of degradation signals in the ubiquitin-proteasome system. Nat. Rev. Mol. Cell Biol. 9, 679-689.
87. Vembar, S. S. and J. L. Brodsky (2008) One step at a time: endoplasmic reticulum-associated degradation. Nat. Rev. Mol. Cell Biol. 9, 944-958.
88. Finley, D., H. D. Ulrich, T. Sommer, and P. Kaiser (2012) The ubiquitin-proteasome system of Saccharomyces cerevisiae. Genetics 192, 319-360.
89. Durairaj, G. and P. Kaiser (2014) The 26S proteasome and initiation of gene transcription. Biomolecules 4, 827-847.
90. Kleiger, G. and T. Mayor (2014) Perilous journey: a tour of the ubiquitin-proteasome system. Trends Cell Biol. 24, 352-359.
91. Greene, E. R., K. C. Dong, and A. Martin (2020) Understanding the 26S proteasome molecular machine from a structural and conformational dynamics perspective. Curr. Opin. Struct. Biol. 61, 33-41.
92. Chen, X., Z. M. Htet, E. López-Alfonzo, A. Martin, and K. J. Walters (2020) Proteasome interaction with ubiquitinated substrates: from mechanisms to therapies. FEBS J. 287, 4-10.
93. Hill, S. and G. Kleiger (2017) Self-regulating ubiquitin ligases. EMBO J. 36, 392-393.
94. Dove, K. K. and R. E. Klevit (2017) RING-between-RING E3 ligases: emerging themes amid the variations. J. Mol. Biol. 429, 3363-3375.
95. Bartel, B. and V. Citovsky (2012) Focus on ubiquitin in plant biology. Plant Physiol. 160, 1.
96. Zattas, D. and M. Hochstrasser (2015) Ubiquitin-dependent protein degradation at the yeast endoplasmic reticulum and nuclear envelope. Crit. Rev. Biochem. Mol. Biol. 50, 1-17.
97. Vittal, V., M. D. Stewart, P. S. Brzovic, and R. E. Klevit (2015) Regulating the regulators: recent revelations in the control of E3 ubiquitin ligases. J. Biol. Chem. 290, 21244-21251.
98. Goo, M. S., S. L. Scudder, and G. N. Patrick (2015) Ubiquitin-dependent trafficking and turnover of ionotropic glutamate receptors. Front. Mol. Neurosci. 8, 60.
99. Finley, D., X. Chen, and K. J. Walters (2016) Gates, channels, and switches: elements of the proteasome machine. Trends Biochem. Sci. 41, 77-93.
100. Shearer, R. F., M. Iconomou, C. K. Watts, and D. N. Saunders (2015) Functional roles of the E3 ubiquitin ligase UBR5 in cancer. Mol. Cancer Res. 13, 1523-1532.
101. Ordureau, A., C. Munch, and J. W. Harper (2015) Quantifying ubiquitin signaling. Mol. Cell 58, 660-676.
102. Brodsky, J. L. and W. R. Skach (2011 ) Protein folding and quality control in the endoplasmic reticulum: Recent lessons from yeast and mammalian cell systems. Curr. Opin. Cell Biol. 23, 464-475.
103. Xie, Y. (2011) Structure, assembly and homeostatic regulation of the 26S proteasome. J. Mol. Cell Biol. 2, 308-317.
104. Schulman, B. A. (2011) Twists and turns in ubiquitin-like conjugation cascades. Prot. Sci. 20, 1941-1954.
105. Hochstrasser, M. (2009) Origin and function of ubiquitin-like proteins. Nature 458, 422-429.
106. Gonda, D. K., A. Bachmair, I. Wünning, J. W. Tobias, W. S. Lane, and A. Varshavsky (1989) Universality and structure of the N-end rule. J. Biol. Chem. 264, 16700-16712.
107. Baker, R. T. and A. Varshavsky (1991) Inhibition of the N-end rule pathway in living cells. Proc. Natl. Acad. Sci. USA 88, 1090-1094.
108. Baker, R. T. and A. Varshavsky (1995) Yeast N-terminal amidase: a new enzyme and component of the N-end rule pathway. J. Biol. Chem. 270, 12065-12074.
109. Dohmen, R. J., K. Madura, B. Bartel, and A. Varshavsky (1991) The N-end rule is mediated by the UBC2 (RAD6) ubiquitin-conjugating enzyme. Proc. Natl. Acad. Sci. USA 88, 7351-7355.
110. Tobias, J. W., T. E. Shrader, G. Rocap, and A. Varshavsky (1991) The N-end rule in bacteria. Science 254, 1374-1377.
111. Johnson, E. S., B. Bartel, W., and A. Varshavsky (1992) Ubiquitin as a degradation signal. EMBO J. 11, 497-505.
112. Johnson, E. S., P. C. Ma, I. M. Ota, and A. Varshavsky (1995) A proteolytic pathway that recognizes ubiquitin as a degradation signal. J. Biol. Chem. 270, 17442-17456.
113. Grigoryev, S., A. E. Stewart, Y. T. Kwon, S. M. Arfin, R. A. Bradshaw, N. A. Jenkins, N. G. Copeland, and A. Varshavsky (1996) A mouse amidase specific for N-terminal asparagine. The gene, the enzyme, and their function in the N-end rule pathway. J. Biol. Chem. 271, 28521-28532.
114. Shrader, T. E., J. W. Tobias, and A. Varshavsky (1993) The N-end rule in Escherichia coli: cloning and analysis of the leucyl, phenylalanyl-tRNA-protein transferase gene aat. J. Bact. 175, 4364-4374.
115. Suzuki, T. and A. Varshavsky (1999) Degradation signals in the lysine-asparagine sequence space. EMBO J. 18, 6017-6026.
116. Turner, G. C. and A. Varshavsky (2000) Detecting and measuring cotranslational protein degradation in vivo. Science 289, 2117-2120.
117. Kwon, Y. T., A. S. Kashina, I. V. Davydov, R.-G. Hu, J. Y. An, J. W. Seo, F. Du, and A. Varshavsky (2002) An essential role of N-terminal arginylation in cardiovascular development. Science 297, 96-99.
118. An, J. Y., J. W. Seo, T. Tasaki, M. J. Lee, A. Varshavsky, and Y. T. Kwon (2006) Impaired neurogenesis and cardiovascular development in mice lacking the E3 ubiquitin ligases UBR1 and UBR2 of the N-end rule pathway. Proc. Natl. Acad. Sci. USA 103, 6212-6217.
119. Hu, R.-G., J. Sheng, Q. Xin, Z. Xu, T. T. Takahashi, and A. Varshavsky (2005) The N-end rule pathway as a nitric oxide sensor controlling the levels of multiple regulators. Nature 437, 981-986.
120. Graciet, E., R. G. Hu, K. Piatkov, J. H. Rhee, E. M. Schwarz, and A. Varshavsky (2006) Aminoacyl-transferases and the N-end rule pathway in a human pathogen. Proc. Natl. Acad. Sci. USA 103, 3078-3083.
121. Hu, R.-G., H. Wang, Z. Xia, and A. Varshavsky (2008) The N-end rule pathway is a sensor of heme. Proc. Natl. Acad. Sci. USA 105, 76-81.
122. Hwang, C. S. and A. Varshavsky (2008) Regulation of peptide import through phosphorylation of Ubr1, the ubiquitin ligase of the N-end rule pathway. Proc. Natl. Acad. Sci. USA 105, 19188-19193.
123. Hwang, C. S., A. Shemorry, and A. Varshavsky (2010) N-terminal acetylation of cellular proteins creates specific degradation signals. Science 327, 973-977.
124. Brower, C. S. and A. Varshavsky (2009) Ablation of arginylation in the mouse N-end rule pathway: loss of fat, higher metabolic rate, damaged spermatogenesis, and neurological perturbations. PLoS ONE 4, e7757.
125. Wang, H., K. I. Piatkov, C. S. Brower, and A. Varshavsky (2009) Glutamine-specific N-terminal amidase, a component of the N-end rule pathway. Mol. Cell 34, 686-695.
126. Hwang, C. S., A. Shemorry, and A. Varshavsky (2010) The N-end rule pathway is mediated by a complex of the RING-type Ubr1 and HECT-type Ufd4 ubiquitin ligases. Nat. Cell Biol. 12, 1177-1185.
127. Hwang, C. S., M. Sukalo, O. Batygin, M. C. Addor, H. Brunner, A. P. Aytes, J. Mayerle, H. K. Song, A. Varshavsky, and M. Zenker (2011) Ubiquitin ligases of the N-end rule pathway: assessment of mutations in UBR1 that cause the Johanson-Blizzard syndrome. PLoS One 6, e24925.
128. Piatkov, K. I., C. S. Brower, and A. Varshavsky (2012) The N-end rule pathway counteracts cell death by destroying proapoptotic protein fragments. Proc. Natl. Acad. Sci. USA 109, E1839-E1847.
129. Piatkov, K. I., L. Colnaghi, M. Bekes, A. Varshavsky, and T. T. Huang (2012) The auto-generated fragment of the Usp1 deubiquitylase is a physiological substrate of the N-end rule pathway. Mol. Cell 48, 926-933.
130. Piatkov, K. I., J.-H. Oh, Y. Liu, and A. Varshavsky (2014) Calpain-generated natural protein fragments as short-lived substrates of the N-end rule pathway. Proc. Natl. Acad. Sci. USA 111, E817-E826.
131. Piatkov, K. I., T. M. Vu, C. S. Hwang, and A. Varshavsky (2015) Formyl-methionine as a degradation signal at the N-termini of bacterial proteins. Microbial Cell 2, 376-393.
132. Park, S. E., J. M. Kim, O. H. Seok, H. Cho, B. Wadas, S. Y. Kim, A. Varshavsky, and C. S. Hwang (2015) Control of mammalian G protein signaling by N-terminal acetylation and the N-end rule pathway. Science 347, 1249-1252.
133. Wadas, B., J. Borjigin, Z. Huang, J.-H. Oh, C.-S. Hwang, and A. Varshavsky (2016) Degradation of serotonin N-acetyltransferase, a circadian regulator, by the N-end rule pathway. J. Biol. Chem. 291, 17178-17196.
134. Wadas, B., K. I. Piatkov, C. S. Brower, and A. Varshavsky (2016) Analyzing N-terminal arginylation through the use of peptide arrays and degradation assays. J. Biol. Chem. 291, 20976-20992.
135. Chen, S. J., X. Wu, B. Wadas, J.-H. Oh, and A. Varshavsky (2017) An N-end rule pathway that recognizes proline and destroys gluconeogenic enzymes. Science 355, 366.
136. Dougan, D. A. and A. Varshavsky (2018) Understanding the Pro/N-end rule pathway. Nat. Chem. Biol. 14, 415-416.
137. Oh, J. H., J. Y. Hyun, and A. Varshavsky (2017) Control of Hsp90 chaperone and its clients by N-terminal acetylation and the N-end rule pathway. Proc. Natl. Acad. Sci. USA 114, E4370-E4379.
138. Kim, J. M., O. H. Seok, S. Ju, J. E. Heo, J. Yeom, D. S. Kim, J. Y. Yoo, A. Varshavsky, C. Lee, and C. S. Hwang (2018) Formyl-methionine as an N-degron of a eukaryotic N-end rule pathway. Science 362, eaat0174.
139. Melnykov, A., S. J. Chen, and A. Varshavsky (2019) Gid10 as an alternative N-recognin of the Pro/N-degron pathway. Proc. Natl. Acad. Sci. USA 116, 15914-15923.
140. Chen, S. J., A. Melnykov, and A. Varshavsky (2020) Evolution of substrates and components of the Pro/N-degron pathway. Biochemistry 59, 541-551.
141. Dong, C., S. J. Chen, A. Melnykov, S. Weirich, K. Sun, A. Jeltsch, A. Varshavsky, and J. Min (2020) Recognition of nonproline N-terminal residues by the Pro/N-degron pathway. Proc. Natl. Acad. Sci. USA 117, 14158-14167.
142. Oh, J. H., J. Y. Hyun, S. J. Chen, and A. Varshavsky (2020) Five enzymes of the Arg/N-degron pathway form a targeting complex: the concept of superchanneling. Proc. Natl. Acad. Sci. USA 117, 10778-10788.
143. Vu, T. T. M. and A. Varshavsky (2020) The ATF3 transcription factor is a short-lived substrate of the Arg/N-degron pathway. Biochemistry 59, 2796-2812.
144. Vu, T. T. M., D. C. Mitchell, S. P. Gygi, and A. Varshavsky (2020) The Arg/N-degron pathway targets transcription factors and regulates specific genes. Proc. Natl. Acad. Sci. USA 117, 31094-31104.
145. Kwon, D. H., O. H. Park, L. Kim, Y. O. Jung, Y. Park, H. Jeong, J. Hyun, Y. K. Kim, and H. K. Song (2018) Insights into degradation mechanism of N-end rule substrates by p62/SQSTM1 autophagy adapter. Nat. Commun. 9, 3291.
146. Yoo, Y. D., S. R. Mun, C. H. Ji, K. W. Sung, K. Y. Kang, A. J. Heo, S. H. Lee, J. Y. An, J. Hwang, X. Q. Xie, A. Ciechanover, B. Y. Kim, and Y. T. Kwon (2018) N-terminal arginylation generates a bimodal degron that modulates autophagic proteolysis. Proc. Natl. Acad. Sci. USA 115, E2716-E2724.
147. Shim, S. M., H. R. Choi, K. W. Sung, Y. J. Lee, S. T. Kim, D. Kim, S. R. Mun, J. Hwang, H. Cha-Molstad, A. Ciechanover, B. Y. Kim, and Y. T. Kwon (2018) The endoplasmic reticulum-residing chaperone BiP is short-lived and metabolized through N-terminal arginylation. Sci. Signal. 11, eaan0630.
148. Kim, S. T., Y. J. Lee, T. Tasaki, J. Hwang, M. J. Kang, E. C. Yi, B. Y. Kim, and Y. T. Kwon (2018) The N-recognin UBR4 of the N-end rule pathway is required for neurogenesis and homeostasis of cell surface proteins. PLoS One 13, e0202260.
149. Ji, C. H., H. Y. Kim, A. J. Heo, S. H. Lee, M. J. Lee, S. B. Kim, G. Srinivasrao, S. R. Mun, H. Cha-Molstad, A. Ciechanover, C. Y. Choi, H. G. Lee, B. Y. Kim, and Y. T. Kwon (2019) The N-degron pathway mediates ER-phagy. Mol. Cell 75, 1058-1072.e1059.
150. Cha-Molstad, H., J. E. Yu, Z. Feng, S. H. Lee, J. G. Kim, P. Yang, B. Han, K. W. Sung, Y. D. Yoo, J. Hwang, T. McGuire, S. M. Shim, H. D. Song, S. Ganipisetti, N. Wang, J. M. Jang, M. J. Lee, S. J. Kim, K. H. Lee, J. T. Hong, A. Ciechanover, I. Mook-Jung, K. P. Kim, X. Q. Xie, Y. T. Kwon, and B. Y. Kim (2017) p62/SQSTM1/sequestosome-1 is an N-recognin of the N-end rule pathway which modulates autophagosome biogenesis. Nat. Commun. 8, 102.
151. Andréasson, C. and P. O. Ljungdahl (2004) The N-terminal regulatory domain of Stp1p is modular and, fused to an artificial transcription factor, confers full SS1--Ptr3p-Ssy5p sensor control. Mol. Cell. Biol. 24, 7503-7513.
152. Omnus, D. J., T. Pfirrmann, C. Andreasson, and P. O. Ljungdahl (2011) A phosphodegron controls nutrient-induced proteasomal activation of the signaling protease Ssy5. Mol. Biol. Cell 22, 2754-2765.
153. Martins, A., A. Ring, D. J. Omnus, S. Heessen, T. Pfirrmann, and P. O. Ljungdahl (2019) Spatial and temporal regulation of the endoproteolytic activity of the SPS-sensor-controlled Ssy5 signaling protease. Mol. Biol. Cell 30, 2709-2720.
154. Ohsumi, Y. (2014) Historical landmarks of autophagy research. Cell Res. 24, 9-23.
155. Gatica, D., V. Lahiri, and D. J. Klionsky (2018) Cargo recognition and degradation by selective autophagy. Nat. Cell Biol. 20, 233-242.
156. Phol, C. and I. Dikic (2019) Cellular quality control by the ubiquitin-proteasome system and autophagy. Science 366, 818-822.
157. Ji, C. H. and Y. T. Kwon (2017) Crosstalk and interplay between the ubiquitin-proteasome system and autophagy. Mol. Cells 40, 441-449.
158. Balchin, D., M. Hayer-Hartl, and F. U. Hartl (2016) In vivo aspects of protein folding and quality control. Science 353, aac4354.
159. Sun, Z. and J. L. Brodsky (2019) Protein quality control in the secretory pathway. J. Cell Biol. 218, 3171-3187.
160. Watson, E. R., N. G. Brown, J. M. Peters, H. Stark, and B. A. Schulman (2019) Posing the APC/C E3 ubiquitin ligase to orchestrate cell division. Trends Cell Biol. 29, 117-134.
161. Gierisch, M. E., T. A. Giovannucci, and N. P. Dantuma (2020) Reporter-based screens for the ubiquitin/proteasome system. Front Chem 8, 64.
162. Schweitzer, A., A. Aufderheide, T. Rudack, F. Beck, G. Pfeifer, J. M. Plitzko, E. Sakata, K. Schulten, F. Forster, and W. Baumeister (2016) Structure of the human 26S proteasome at a resolution of 3.9 A. Proc. Natl. Acad. Sci USA 113, 7816-7821.
163. Finley, D. and M. A. Prado (2020) The proteasome and its network: engineering for adaptability. Cold Spring Harb. Perspect. Biol. 12, a033985.
164. Collins, G. A. and A. L. Goldberg (2017) The logic of the 26S proteasome. Cell 169, 792-806.
165. Budenholzer, L., C. L. Cheng, Y. Li, and M. Hochstrasser (2017) Proteasome structure and assembly. J. Mol. Biol. 429, 3500-3524.
166. Yu, H. and A. Matouschek (2017) Recognition of client proteins by the proteasome. Annu. Rev. Biophys. 46, 149-173.
167. Dougan, D. A., D. Micevski, and K. N. Truscott (2011) The N-end rule pathway: from recognition by N-recognins to destruction by AAA+ proteases. Biochim. Biophys. Acta 1823, 83-91.
168. Tasaki, T. S., S. M. Sriram, K. S. Park, and Y. T. Kwon (2012) The N-end rule pathway. Annu. Rev. Biochem. 81, 261-289.
169. Aksnes, H., A. Drazic, M. Marie, and T. Arnesen (2016) First things first: vital protein marks by N-terminal acetyltransferases. Trends Biochem. Sci. 9, 746-760.
170. Gibbs, D. J., J. Bacardit, A. Bachmair, and M. J. Holdsworth (2014) The eukaryotic N-end rule pathway: conserved mechanisms and diverse functions. Trends Cell Biol. 24, 603-611.
171. Dissmeyer, N., S. Rivas, and E. Graciet (2017) Life and death of proteins after protease cleavage: protein degradation by the N-end rule pathway. New Phytol. 218, 929-935.
172. Dong, C., H. Zhang, L. Li, W. Tempel, P. Loppnau, and J. Min (2018) Molecular basis of GID-mediated recognition of degrons for the Pro/N-end rule pathway. Nat. Chem. Biol. 14, 466-473.
173. Brower, C. S., K. I. Piatkov, and A. Varshavsky (2013) Neurodegeneration-associated protein fragments as short-lived substrates of the N-end rule pathway. Mol. Cell 50, 161-171.
174. Kasu, Y. A. T., S. Alemu, A. Lamari, N. Loew, and C. S. Brower (2018) The N-termini of TAR DNA-binding protein 43 (TDP43) C-terminal fragments influence degradation, aggregation propensity, and morphology. Mol. Cell. Biol. 38, e00243-00218.
175. Kim, H. K., R. R. Kim, J. H. Oh, H. Cho, A. Varshavsky, and C. S. Hwang (2014) The N-terminal methionine of cellular proteins as a degradation signal. Cell 156, 158-169.
176. Nguyen, K. T., C. S. Lee, S. H. Mun, T. T. Nhung, S. K. Park, and C. S. Hwang (2018) N-terminal acetylation and N-end rule pathway control degradation of the lipid droplet protein PLIN2. J. Biol. Chem. 294, 379-388.
177. Shemorry, A., C. S. Hwang, and A. Varshavsky (2013) Control of protein quality and stoichiometries by N-terminal acetylation and the N-end rule pathway. Mol. Cell 50, 540-551.
178. Rivera-Rivera, I., G. Román-Hernández, R. T. Sauer, and T. A. Baker (2014) Remodeling of a delivery complex allows ClpS-mediated degradation of N-degron substrates. Proc. Natl. Acad. Sci. USA 111, E3853-E3859.
179. Vicente, J., G. M. Mendiondo, M. Movahedi, M. Peirats-Llobet, Y. T. Juan, Y. Y. Shen, C. Dambire, K. Smart, P. L. Rodriguez, Y. Y. Charng, J. E. Gray, and M. J. Holdsworth (2017) The Cys-Arg/N-end rule pathway is a general sensor of abiotic stress in flowering plants. Curr. Biol. 27, 3183-3190.e3184.
180. Oh, J. H., S. J. Chen, and A. Varshavsky (2017) A reference-based protein degradation assay without global translation inhibitors. J. Biol. Chem. 292, 21457-21465.
181. Masson, N., T. P. Keeley, B. Giuntoli, M. D. White, M. L. Puerta, P. Perata, R. J. Hopkinson, E. Flashman, F. Licausi, and P. J. Ratcliffe (2019) Conserved N-terminal cysteine dioxygenases transduce responses to hypoxia in animals and plants. Science 365, 65-69.
182. Gao, X., J. Yeom, and E. A. Groisman (2019) The expanded specificity and physiological role of a widespread N-degron recognin. Proc. Natl. Acad. Sci. USA 116, 18629-18637.
183. Timms, R. T., Z. Zhang, D. Y. Rhee, J. W. Harper, I. Koren, and S. J. Elledge (2019) A glycine-specific N-degron pathway mediates the quality control of protein N-myristoylation. Science 365, eaaw4912.
184. Park, J. S., J. Y. Lee, Y. T. K. Nguyen, N. W. Kang, E. K. Oh, D. M. Jang, H. J. Kim, D. D. Kim, and B. W. Han (2020) Structural analyses on the deamidation of N-terminal Asn in the human N-degron pathway. Biomolecules 10, 163.
185. Qiao, S., C. R. Langlois, J. Chrustowicz, D. Sherpa, O. Karayel, F. M. Hansen, V. Beier, S. von Gronau, D. Bollschweiler, T. Schafer, A. F. Alpi, M. Mann, J. R. Prabu, and B. A. Schulman (2020) Interconversion between anticipatory and active GID E3 ubiquitin ligase conformations via substrate receptor assembly. Mol. Cell 77, 150-163.e159.
186. Inobe, T., S. Fishbain, S. Prakash, and A. Matouschek (2011) Defining the geometry of the two-component proteasome degron. Nat. Chem. Biol. 7, 161-167.
187. Kwon, Y. T., S. A. Balogh, I. V. Davydov, A. S. Kashina, J. K. Yoon, Y. Xie, A. Gaur, L. Hyde, V. H. Denenberg, and A. Varshavsky (2000) Altered activity, social behavior, and spatial memory in mice lacking the NTAN1 amidase and the asparagine branch of the N-end rule pathway. Mol. Cell. Biol. 20, 4135-4148.
188. Olivares, A. O., T. A. Baker, and R. T. Sauer (2018) Mechanical protein unfolding and degradation. Annu. Rev. Physiol. 80, 413-429.
189. Eldeeb, M. A. and R. P. Fahlman (2014) The anti-apoptotic form of tyrosine kinase Lyn that is generated by proteolysis is degraded by the N-end rule pathway. Oncotarget 5, 2714-2722.
190. Gibbs, D. J. and M. J. Holdsworth (2020) Every breath you take: new insights into plant and animal oxygen sensing. Cell 180, 22-24.
191. Weaver, B. P., Y. M. Weaver, S. Mitani, and M. Han (2017) Coupled caspase and N-end rule ligase activities allow recognition and degradation of pluripotency factor LIN-28 during non-apoptotic development. Dev. Cell 41, 665-673.e666.
192. Nillegoda, N. B., M. A. Theodoraki, A. K. Mandal, K. J. Mayo, H. Y. Ren, R. Sultana, K. Wu, J. Johnson, D. M. Cyr, and A. J. Caplan (2010) Ubr1 and Ubr2 function in a quality control pathway for degradation of unfolded cytosolic proteins. Mol. Biol. Cell 21, 2102-2116.
193. Varshavsky, A. (2004) Spalog and sequelog: neutral terms for spatial and sequence similarity. Curr. Biol. 14, R181-R183.
194. Kwon, Y. T., Z. Xia, I. V. Davydov, S. H. Lecker, and A. Varshavsky (2001) Construction and analysis of mouse strains lacking the ubiquitin ligase UBR1 (E3-alpha) of the N-end rule pathway. Mol. Cell. Biol. 21, 8007-8021.
195. Kwon, Y. T., Z. X. Xia, J. Y. An, T. Tasaki, I. V. Davydov, J. W. Seo, Y. Xie, and A. Varshavsky (2003) Female lethality and apoptosis of spermatocytes in mice lacking the UBR2 ubiquitin ligase of the N-end rule pathway. Mol. Cell. Biol. 23, 8255-8271.
196. Yamano, K. and R. J. Youle (2013) PINK1 is degraded through the N-end rule pathway. Autophagy 9, 1758-1769.
197. Choi, W. S., B.-C. Jeong, Y. J. Joo, M.-R. Lee, J. Kim, M. J. Eck, and H. K. Song (2010) Structural basis for the recognition of N-end rule substrates by the UBR box of ubiquitin ligases. Nat. Struct. Mol. Biol. 17, 1175-1181.
198. Matta-Camacho, E., G. Kozlov, F. F. Li, and K. Gehring (2010) Structural basis of substrate recognition and specificity in the N-end rule pathway. Nat. Struct. Mol. Biol. 17, 1182-1188.
199. Kim, M. K., S. J. Oh, B. G. Lee, and H. K. Song (2016) Structural basis for dual specificity of yeast N-terminal amidase in the N-end rule pathway. Proc. Natl. Acad. Sci. USA 113, 12438-12443.
200. Keiler, K. C., P. R. Waller, and R. T. Sauer (1996) Role of a peptide tagging system in degradation of proteins synthesized from damaged messenger RNA. Science 271, 990-993.
201. Koren, I., R. T. Timms, T. Kula, Q. Xu, M. Z. Li, and S. J. Elledge (2018) The eukaryotic proteome is shaped by E3 ubiquitin ligases targeting C-terminal degrons. Cell 173, 1622-1635.e1614.
202. Lin, H. C., C. W. Yeh, Y. F. Chen, T. T. Lee, P. Y. Hsieh, D. V. Rusnac, S. Y. Lin, S. J. Elledge, N. Zheng, and H. S. Yen (2018) C-terminal end-directed protein elimination by CRL2 ubiquitin ligases. Mol. Cell 70, 602-613.e603.
203. Chatr-Aryamontri, A., A. van der Sloot, and M. Tyers (2018) At long last, a C-terminal bookend for the ubiquitin code. Mol. Cell 70, 568-571.
204. Goldknopf, I. A. and H. Busch (1977) Isopeptide linkage between nonhistone and histone 2A polypeptides of chromosomal conjugate-protein A24. Proc. Natl. Acad. Sci. USA 74, 864-868.
205. Schlesinger, D. H., G. Goldstein, and H. D. Niall (1975) The complete amino acid sequence of ubiquitin, an adenylate cyclase stimulating polypeptide probably universal in living cells. Biochemistry 14, 2214-2218.
206. Bakayev, V. V., A. A. O. Melnickov, V.D., and A. Varshavsky (1975) Isolation of chromatin subunits. Nucl. Acids Res. 2, 1401-1419.
207. Varshavsky, A., V. V. Bakayev, and G. P. Georgiev (1976) Heterogeneity of chromatin subunits in vitro and location of histone H1. Nucl. Acids Res. 3, 477-492.
208. Levinger, L., J. Barsoum, and A. Varshavsky (1981) Two-dimensional hybridization mapping of nucleosomes. J. Mol. Biol. 146, 287-304.
209. Levinger, L. and A. Varshavsky (1982) Selective arrangement of ubiquitinated and D1 protein-containing nucleosomes within the Drosophila genome. Cell 28, 375-385.
210. Levinger, L. and A. Varshavsky (1980) Separation of nucleosomes containing and lacking ubiquitin-H2A semihistone. Proc. Natl. Acad. Sci. USA 77, 3244-3248.
211. Balzi, E., M. Choder, W. Chen, A. Varshavsky, and A. Goffeau (1990) Cloning and functional analysis of the arginyl-tRNA-protein transferase gene ATE1 of Saccharomyces cerevisiae. J. Biol. Chem. 265, 7464-7471.
212. Hochstrasser, M., M. J. Ellison, V. Chau, and A. Varshavsky (1991) The short-lived MATalpha2 transcriptional regulator is ubiquitinated in vivo. Proc. Natl. Acad. Sci. USA 88, 4606-4610.
213. Ciechanover, A., S. Elias, H. Heller, and A. Hershko (1982) "Covalent affinity" purification of ubiquitin-activating enzyme. J. Biol. Chem. 257, 2537-2542.
214. Hershko, A., H. Heller, S. Elias, and A. Ciechanover (1983) Components of ubiquitin-protein ligase system. Resolution, affinity purification, and role in protein breakdown. J. Biol. Chem. 258, 8206-8214.
215. Hershko, A., E. Leshinsky, D. Ganoth, and H. Heller (1984) ATP-dependent degradation of ubiquitin-protein conjugates. Proc. Natl. Acad. Sci. USA 81, 1619-1623.
216. Wilkinson, K. D., M. K. Urban, and A. L. Haas (1980) Ubiquitin is the ATP-dependent proteolytic factor of rabbit reticulocytes. J. Biol. Chem. 255, 7529-7532.
217. Marunouchi, T., S. Mita, Y. Matsumoto, and M. Yamada (1980) Disappearance of a chromosomal basic protein from cells of a mouse temperature-sensitive mutant defective in histone phosphorylation. Biochem. Biophys. Res. Commun. 95, 126-131.
218. Matsumoto, Y., H. Yasuda, T. Marunouchi, H. Yamamoto, and M. Yamada (1983) Decrease in uH2A (protein A24) in a mouse temperature-sensitive mutant. FEBS Lett. 151, 139-142.
219. Evans, T., E. T. Rosenthal, J. Youngblom, D. Distel, and T. Hunt (1983) Cyclin: a protein specified by maternal mRNA in sea urchin eggs that is destroyed at each cleavage division. Cell 33, 389-396.
220. Glotzer, M., A. W. Murray, and M. Kirschner (1991) Cyclin is degraded by the ubiquitin pathway. Nature 349, 132-138.
221. Hershko, A., D. Ganoth, J. Pehrson, R. E. Palazzo, and L. H. Cohen (1991) Methylated ubiquitin inhibits cyclin degradation in clam embryo extracts. J. Biol. Chem. 266, 16376-16379.
222. Rumpf, S. and S. Jentsch (2006) Functional division of substrate processing cofactors of the ubiquitin-selective Cdc48 chaperone. Mol. Cell 21, 261-269.
223. Erbse, A., R. Schmidt, T. Bornemann, J. Schneider-Mergener, A. Mogk, R. Zahn, D. A. Dougan, and B. Bukau (2006) ClpS is an essential component of the N-end rule pathway in Escherichia coli. Nature 439, 753-756.
224. Mogk, A., R. Schmidt, and B. Bukau (2007) The N-end rule pathway of regulated proteolysis: prokaryotic and eukaryotic strategies. Trends Cell Biol. 17, 165-172.
225. Yeom, J., K. J. Wayne, and E. A. Groisman (2017) Sequestration from protease adaptor confers differential stability to protease substrate. Mol. Cell 66, 234-246.e235.
226. Yeom, J., X. Gao, and E. A. Groisman (2018) Reduction in adaptor amounts establishes degradation hierarchy among protease substrates. Proc. Natl. Acad. Sci. USA 115, E4483-e4492.
227. Wang, K. H., G. Roman-Hernandez, R. A. Grant, T. T. Sauer, and T. A. Baker (2008) The molecular basis of N-end rule recognition. Mol. Cell 32, 406-414.
228. Wang, K. H., E. S. C. Oakes, R. T. Sauer, and T. A. Baker (2008) Tuning the strength of a bacterial N-end rule signal. J. Biol. Chem. 283, 24600-24607.
229. Stein, B. J., R. A. Grant, R. T. Sauer, and T. A. Baker (2016) Structural basis of an N-degron adaptor with more stringent specificity. Structure 24, 232-242.
230. Román-Hernández, G., J. Y. Hou, R. A. Grant, R. T. Sauer, and T. A. Baker (2011) The ClpS adaptor mediates staged delivery of N-end rule substrates to the AAA+ ClpAP protease. Mol. Cell 43, 217-228.
231. Román-Hernández, G., R. A. Grant, R. T. Sauer, and T. A. Baker (2009) Molecular basis of substrate selection by the N-end rule adaptor protein ClpS. Proc. Natl. Acad. Sci. USA 106, 8888-8893.
232. Ninnis, R. L., S. K. Spall, G. H. Talbo, K. N. Truscott, and D. A. Dougan (2009) Modification of PATase by L/F-transferase generates a ClpS-dependent N-end rule substrate in Escherichia coli. EMBO J. 28, 1732-1744.
233. Humbard, M. A., S. Surkov, G. M. De Donatis, L. Jenkins, and M. R. Maurizi (2013) The N-degradome of Escherichia coli: limited proteolysis in vivo generates a large pool of proteins bearing N-degrons. J. Biol. Chem. 288, 28913-28924.
234. Tomita, T. and A. Matouschek (2019) Substrate selection by the proteasome through initiation regions. Protein Sci. 28, 1222-1232.
235. Inobe, T. and A. Matouschek (2014) Paradigms of protein degradation by the proteasome. Curr. Op. Struct. Biol. 24, 156-164.
236. Kaji, H., G. D. Novelli, and A. Kaji (1963) A soluble amino acid-incorporating system from rat liver. Biochim. Biophys. Acta 76, 474-479.
237. Soffer, R. L., Biochemistry and biology of aminoacyl-tRNA-protein transferases., in Transfer RNA: Biological Aspects., D. Söll, J. Abelson, and P.R. Schimmel, Editors. 1980, Cold Spring Harbor Laboratory Press: Cold Spring Harbor, NY. p. 493-505.
238. Ferber, S. and A. Ciechanover (1987) Role of arginine-tRNA in protein degradation by the ubiquitin pathway. Nature 326, 808-811.
239. Hicke, L. and H. Riezman (1996) Ubiquitination of a yeast plasma membrane receptor signals its ligand-stimulated endocytosis. Cell 84, 277-287.
240. Komander, D. and M. Rape (2012) The ubiquitin code. Annu. Rev. Biochem. 81, 203-229.
241. Mena, E. L., R. A. S. Kjolby, R. A. Saxton, A. Werner, B. G. Lew, J. M. Boyle, R. Harland, and M. Rape (2018) Dimerization quality control ensures neuronal development and survival. Science 362, eaap8236.
242. Kerscher, O., R. Felberbaum, and M. Hochstrasser (2006) Modification of proteins by ubiquitin and ubiquitin-like proteins. Annu. Rev. Cell Dev. Biol. 22, 159-180.
243. Peters, J.-M. (2006) The anaphase-promoting complex/cyclosome: a machine designed to destroy. Nat. Rev. Mol. Cell Biol. 7, 644-656.
244. Harper, J. W. and B. A. Schulman (2006) Structural complexity in ubiquitin recognition. Cell 124, 1133-1336.
245. Rubinsztein, D. C. (2006) The roles of intracellular protein-degradation pathways in neurodegeneration. Nature 443, 780-786.
246. Mukhopadhyay, D. and H. Reizman (2007) Proteasome-independent functions of ubiquitin in endocytosis and signaling. Science 315, 201-205.
247. Hunter, T. (2007) The age of crosstalk: phosphorylation, ubiquitination, and beyond. Mol. Cell 28, 730-738.
248. Demartino, G. N. and T. G. Gillette (2007) Proteasomes: machines for all reasons. Cell 129, 747-759.
249. Hanna, J. and D. Finley (2007) A proteasome for all occasions. FEBS Lett. 581, 2854-2861.
250. Yi, J. J. and M. D. Ehlers (2007) Emerging roles for ubiquitin and protein degradation in neuronal function. Pharmacol. Rev. 59, 14-39.
251. Geiss-Friedlander, R. and F. Melchior (2007) Concepts in sumoylation: a decade on. Nat. Rev. Mol. Cell Biol. 8, 947-956.
252. Woelk, T., S. Sigismund, L. Penengo, and S. Polo (2007) The ubiquitination code: a signalling problem. Cell Division 2, doi: 10.1186/1747-1028-1182-1111.
253. Inobe, T. and A. Matouschek (2008) Protein targeting to ATP-dependent proteases. Curr. Opin. Struct. Biol. 18, 43-51.
254. Ghislain, M., R. J. Dohmen, F. Levy, and A. Varshavsky (1996) Cdc48p interacts with Ufd3p, a WD repeat protein required for ubiquitin-mediated proteolysis in Saccharomyces cerevisiae. EMBO. J. 15, 4884-4899.
255. Cooney, I., H. Han, M. G. Stewart, R. H. Carson, D. T. Hansen, J. H. Iwasa, J. C. Price, C. P. Hill, and P. S. Shen (2019) Structure of the Cdc48 segregase in the act of unfolding an authentic substrate. Science 365, 502-505.
256. Godderz, D., C. Heinen, F. P. Marchese, T. Kurz, K. Acs, and N. P. Dantuma (2015) Cdc48-independent proteasomal degradation coincides with a reduced need for ubiquitylation. Sci. Rep. 5, 7615.
257. Xia, D., W. K. Tang, and Y. Ye (2016) Structure and function of the AAA+ ATPase p97/Cdc48p. Gene 583, 64-77.
258. Ditzel, M., R. Wilson, T. Tenev, A. Zachariou, A. Paul, E. Deas, and P. Meier (2003) Degradation of DIAP1 by the N-end rule pathway is essential for regulating apoptosis. Nat. Cell Biol. 5, 467-473.
259. Wang, Z., X. Xia, X. Yang, X. Zhang, Y. Liu, D. Wu, Y. Fang, Y. Liu, J. Xu, Y. Qiu, and X. Zhou (2017) A picorna-like virus suppresses the N-end rule pathway to inhibit apoptosis. eLife 6, e30590.
260. Park, M. S., E. Bitto, K. R. Kim, C. A. Bingman, M. D. Miller, H. J. Kim, B. W. Han, and G. N. Phillips, Jr. (2014) Crystal structure of human protein N-terminal glutamine amidohydrolase, an initial component of the N-end rule pathway. PLoS One 9, e111142.
261. Villamil, M. A., J. Chen, Q. Liang, and Z. Zhuang (2012) A noncanonical cysteine protease USP1 is activated through active site modification by USP1-associated factor 1. Biochemistry 51, 2829-2839.
262. Huang, T. T., S. M. B. Nijman, K. D. Mirchandani, P. J. Galardy, M. A. Cohn, W. Haas, S. P. Gygi, H. P. Ploegh, R. Bernards, and A. D. D'Andrea (2006) Regulation of monoubiquitinated PCNA by DUB autocleavage. Nat. Cell Biol. 8, 339-347.
263. Cohn, M. A., P. Kowal, K. Yang, W. Haas, T. T. Huang, S. P. Gygi, and A. D. D'Andrea (2007) A UAF1-containing multisubunit complex regulates the Fanconi anemia pathway. Mol. Cell 28, 786-797.
264. Coleman, K. E., Y. Yin, S. K. L. Lui, S. Keegan, D. Fenyo, D. J. Smith, E. Rothenberg, and T. T. Huang (2022) USP1-trapping lesions as a source of DNA replication stress and genomic instability. Nat. Commun. 13, 1740.
265. Vicente, J., G. M. Mendiondo, J. Pauwels, V. Pastor, Y. Izquierdo, C. Naumann, M. Movahedi, D. Rooney, D. J. Gibbs, K. Smart, A. Bachmair, J. E. Gray, N. Dissmeyer, C. Castresana, R. V. Ray, K. Gevaert, and M. J. Holdsworth (2019) Distinct branches of the N-end rule pathway modulate the plant immune response. New Phytol. 221, 988-1000.
266. de Marchi, R., M. Sorel, B. Mooney, I. Fudal, K. Goslin, K. Kwasniewska, P. T. Ryan, M. Pfalz, J. Kroymann, S. Pollmann, A. Feechan, F. Wellmer, S. Rivas, and E. Graciet (2016) The N-end rule pathway regulates pathogen responses in plants. Sci. Rep. 6, 26020.
267. Kwon, Y. T., A. S. Kashina, and A. Varshavsky (1999) Alternative splicing results in differential expression, activity, and localization of the two forms of arginyl-tRNA-protein transferase, a component of the N-end rule pathway. Mol. Cell. Biol. 19, 182-193.
268. Hu, R.-G., C. S. Brower, H. Wang, I. V. Davydov, J. Sheng, J. Zhou, Y. T. Kwon, and A. Varshavsky (2006) Arginyl-transferase, its specificity, putative substrates, bidirectional promoter, and splicing-derived isoforms. J. Biol. Chem. 281, 32559-32573.
269. Grumati, P. and I. Dikic (2018) Ubiquitin signaling and autophagy. J. Biol. Chem. 293, 5404-5413.
270. Lee, M. J., T. Tasaki, K. Moroi, J. Y. An, S. Kimura, I. V. Davydov, and Y. T. Kwon (2005) RGS4 and RGS5 are in vivo substrates of the N-end rule pathway. Proc. Natl. Acad. Sci. USA 102, 15030-15035.
271. White, M. D., M. Klecker, R. J. Hopkinson, D. A. Weits, C. Mueller, C. Naumann, R. O'Neill, J. Wickens, J. Yang, J. C. Brooks-Bartlett, E. F. Garman, T. N. Grossmann, N. Dissmeyer, and E. Flashman (2017) Plant cysteine oxidases are dioxygenases that directly enable arginyl transferase-catalysed arginylation of N-end rule targets. Nat Commun 8, 14690.
272. Brower, C. S., C. E. Rosen, R. H. Jones, B. C. Wadas, K. I. Piatkov, and A. Varshavsky (2014) Liat1, an arginyltransferase-binding protein whose evolution among primates involved changes in the numbers of its 10-residue repeats. Proc. Natl. Acad. Sci. USA 111, E4936-4945.
273. Arva, A., Y. A. T. Kasu, J. Duncan, M. A. Alkhatatbeh, and C. S. Brower (2021) The Ligand of Ate1 is intrinsically disordered and participates in nucleolar phase separation regulated by Jumonji Domain Containing 6. Proc. Natl. Acad. Sci. USA 118, e2015887118.
274. Hwang, C. S., A. Shemorry, and A. Varshavsky (2009) Two proteolytic pathways regulate DNA repair by cotargeting the Mgt1 alkylguanine transferase. Proc. Natl. Acad. Sci. USA 106, 2142-2147.
275. Zenker, M., J. Mayerle, M. M. Lerch, A. Tagariello, K. Zerres, P. R. Durie, M. Beier, G. Hülskamp, C. Guzman, H. Rehder, F. A. Beemer, B. Hamel, P. Vanlieferinghen, R. Gershoni-Baruch, M. W. Vieira, M. Dumic, R. Auslender, V. L. Gil-da-Silva-Lopes, S. Steinlicht, R. Rauh, S. A. Shalev, C. Thiel, A. Winterpacht, Y. T. Kwon, A. Varshavsky, and A. Reis (2005) Deficiency of UBR1, a ubiquitin ligase of the N-end rule pathway, causes pancreatic dysfunction, malformations and mental retardation (Johanson-Blizzard syndrome). Nat. Genet. 37, 1345-1350.
276. Xie, Y. and A. Varshavsky (2000) Physical association of ubiquitin ligases and the 26S proteasome. Proc. Natl. Acad. Sci. USA 97, 2497-2502.
277. Xie, Y. and A. Varshavsky (2002) UFD4 lacking the proteasome-binding region catalyses ubiquitination but is impaired in proteolysis. Nature Cell Biol. 4, 1003-1007.
278. Steiner, H.-Y., F. Naider, and J. M. Becker (1995) The PTR family: a new group of peptide transporters. Mol. Microbiol. 16, 825-834.
279. Knight, S. A. B., K. T. Tamai, D. J. Kosman, and D. J. Thiele (1994) Identification and analysis of a Saccharomyces cerevisiae copper homeostasis gene encoding a homeodomain protein. Mol. Cell. Biol. 14, 7792-7804.
280. Zhou, P. and D. J. Thiele (1993) Copper and gene regulation in yeast. Biofactors 4, 105-115.
281. Kitamura, K. and H. Fujiwara (2013) The type-2 N-end rule peptide recognition activity of Ubr11 ubiquitin ligase is required for the expression of peptide transporters. FEBS Lett. 587, 214-219.
282. Pan, M., Q. Zheng, T. Wang, L. Liang, J. Mao, C. Zuo, R. Ding, H. Ai, Y. Xie, D. Si, Y. Yu, L. Liu, and M. Zhao (2021) Structural insights into Ubr1-mediated N-degron polyubiquitination. Nature 600, 334-338.
283. Scott, D. C., J. K. Monda, E. J. Bennett, J. W. Harper, and B. A. Schulman (2011) N-terminal acetylation acts as an avidity enhancer within an interconnected multiprotein complex. Science 334, 674-678.
284. Chen, S. J., L. Kim, H. K. Song, and A. Varshavsky (2021) Aminopeptidases trim Xaa-Pro proteins, initiating their degradation by the Pro/N-degron pathway. Proc. Natl. Acad. Sci. USA 118, e2115430118.
285. Roninson, I., H. T. Abelson, D. E. Housman, N. Howell, and A. Varshavsky (1984) Amplification of specific DNA sequences correlates with multidrug resistance in Chinese hamster cells. Nature 309, 626-628.
286. Varshavsky, A. (1981) Phorbol ester dramatically increases incidence of methotrexate-resistant mouse cells: possible mechanisms and relevance to tumor promotion. Cell 25, 561-572.
287. Barsoum, J. and A. Varshavsky (1983) Mitogenic hormones and tumor promoters greatly increase the incidence of cells bearing amplified dihydrofolate reductase genes. Proc. Natl. Acad. Sci. USA 80, 5330-5334.
288. Alfageme, C. R., G. T. Rudkin, and L. H. Cohen (1980) Isolation, properties and cellular distribution of D1, a chromosomal protein of Drosophila. Chromosoma 78, 1-31.
289. Levinger, L. and A. Varshavsky (1982) Protein D1 preferentially binds A+T-rich DNA in vitro and is a component of Drosophila melanogaster nucleosomes containing A+T-rich satellite DNA. Proc. Natl. Acad. Sci. USA 79, 7152-7156.
290. Aulner, N., C. Monod, G. Mandicourt, D. Jullien, O. Cuvier, A. Sall, S. Janssen, U. K. Laemmli, and E. Käs (2002) The AT-hook protein D1 is essential for Drosophila melanogaster development and is implicated in position-effect variegation. Mol. Cell. Biol. 22, 1218-1232.
291. Blattes, R., C. Monod, G. Susbielle, O. Cuvier, J.-H. Wu, T.-S. Hsieh, U. K. Laemmli, and E. Käs (2006) Displacement of D1, HP1 and topoisomerase II from satellite heterochromatin by a specific polyamide. EMBO J. 25, 2397-2408.
292. Strauss, F. and A. Varshavsky (1984) A protein binds to a satellite DNA repeat at three sites which would be brought into proximity by DNA folding in the nucleosome. Cell 37, 889-901.
293. Winter, E. and A. Varshavsky (1989) A DNA binding protein that recognizes oligo(dA)-oligo(dT) tracts. EMBO J. 8, 1867-1877.
294. Zada, D., Y. Sela, N. Matosevich, A. Monsonego, T. Lerer-Goldshtein, Y. Nir, and L. Appelbaum (2021) Parp1 promotes sleep, which enhances DNA repair in neurons. Mol. Cell 81, 4979-4993.e4977.
295. Fried, M. G. and D. M. Crothers (1981) Equilibria and kinetics of lac repressor-operator interactions by polyacrylamide electrophoresis. Nucl. Acids Res. 9, 6505-6525.
296. Garner, M. and A. Revzin (1981) A gel electrophoresis method for quantying the binding of proteins to specific DNA regions: application to components of the Escherichia coli lactose operone regulatory system. Nucl. Acids Res. 9, 3047-3060.
297. Varshavsky, A. (1987) Electrophoretic assay for DNA-binding proteins. Meth. Enzymol. 151, 551-565.
298. Solomon, M. J., P. L. Larsen, and A. Varshavsky (1988) Mapping protein-DNA interactions in vivo with formaldehyde. Cell 53, 937-947.
299. Varshavsky, A. and Y. V. Ilyin (1974) Salt treatment of chromatin induces redistribution of histones. Biochim. Biophys. Acta 340, 207-217.
300. Bartel, B. and A. Varshavsky (1988) Hypersensitivity to heavy water: a new conditional phenotype. Cell 52, 935-941.
301. Dohmen, R. J., P. Wu, and A. Varshavsky (1994) Heat-inducible degron: a method for constructing temperature-sensitive mutants. Science 263, 1273-1276.
302. Varshavsky, A. (2005) Ubiquitin fusion technique and related methods. Meth. Enzymol. 399, 777-799.
303. Lévy, F., N. Johnsson, T. Rümenapf, and A. Varshavsky (1996) Using ubiquitin to follow the metabolic fate of a protein. Proc. Natl. Acad. Sci. USA 93, 4907-4912.
304. Dohmen, R. J. and A. Varshavsky (2005) Heat-inducible degron and the making of conditional mutants. Meth. Enzymol. 399, 799-822.
305. Johnsson, N. and A. Varshavsky (1994) Ubiquitin-assisted dissection of protein transport across cell membranes. EMBO J. 13, 2686-2698.
306. Dunnwald, M., A. Varshavsky, and N. Johnsson (1999) Detection of transient in vivo interactions between substrate and transporter during protein translocation into the endoplasmic reticulum. Mol. Biol. Cell 10, 329-344.
307. Wittke, S., M. Dunnwald, M. Albertsen, and N. Johnsson (2002) Recognition of a subset of signal sequences by Ssh1p, a Sec61p-related protein in the membrane of endoplasmic reticulum of yeast Saccharomyces cerevisiae. Mol. Biol. Cell 13, 2223-2232.
308. Gisler, S. M., S. Kittanakom, D. Fuster, V. Wong, M. Bertic, T. Radanovic, R. A. Hall, H. Murer, J. Biber, D. Markovich, O. W. Moe, and I. Stagljar (2008) Monitoring protein-protein interactions between the mammalian integral membrane transporters and PDZ-interacting partners using a modified split-ubiquitin membrane yeast two-hybrid system. Mol. Cell. Proteomics 7, 1362-1377.
309. Tarassov, K., V. Messier, C. R. Landry, S. Radinovic, M. M. Molina, I. Shames, Y. Malitskaya, J. Vogel, H. Bussey, and S. W. Michnick (2008) An in vivo map of the yeast protein interactome. Science 320, 1465-1470.
310. Kerppola, T. K. (2008) Bimolecular fluorescence complementation (BiFC) analysis as a probe of protein interactions in living cells. Annu. Rev. Biophys. 37, 465-487.
311. Kötter, P., J. E. Weigand, B. Meyer, K.-D. Entian, and B. Suess (2009) A fast and efficient translational control system for conditional expression of yeast genes. Nucl. Ac. Res. 37, e120.
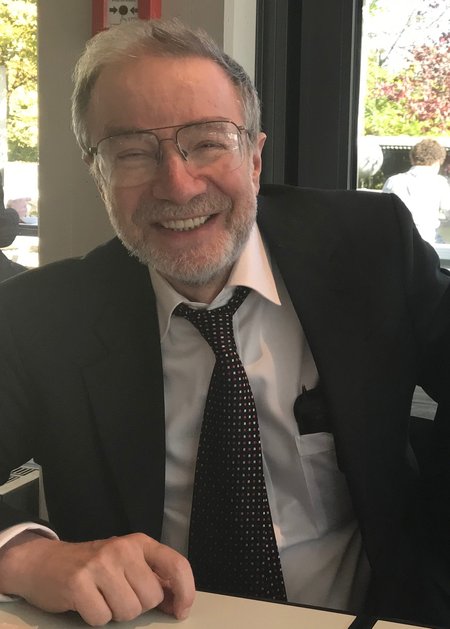
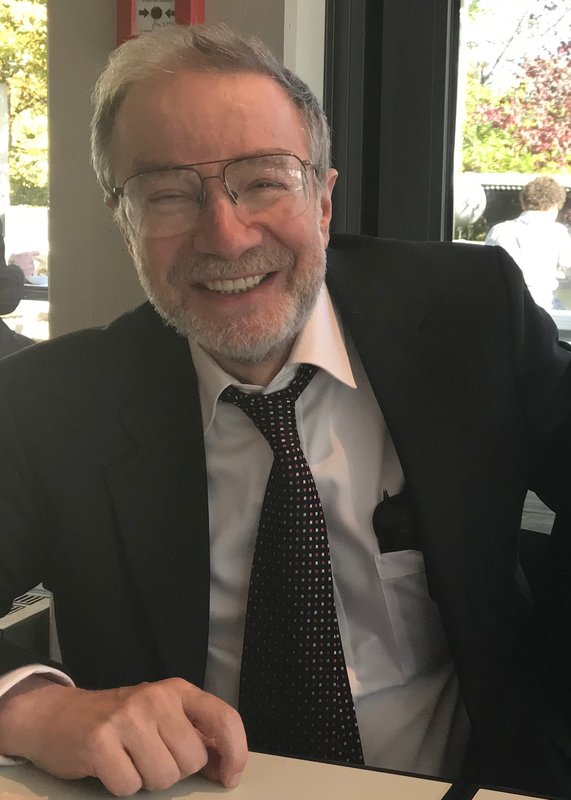
Images on this page:
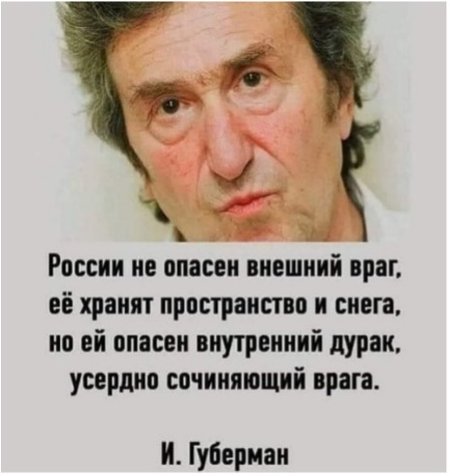
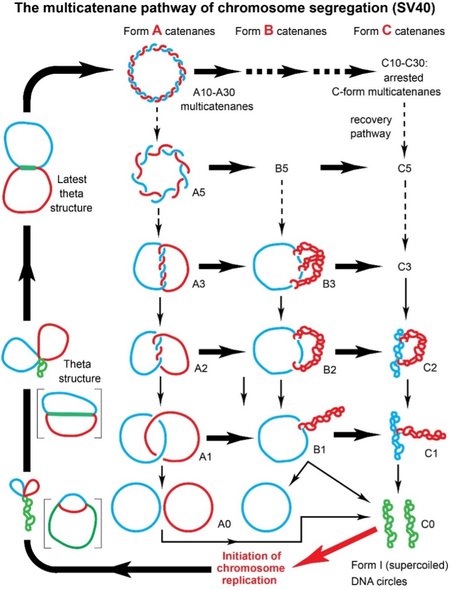
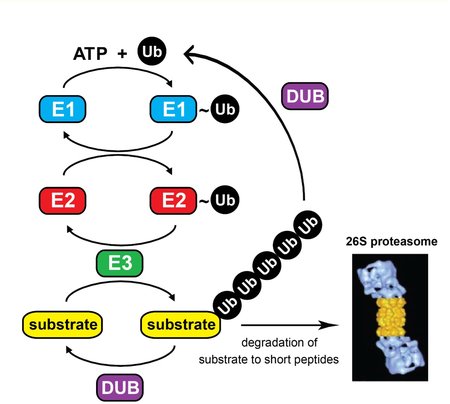
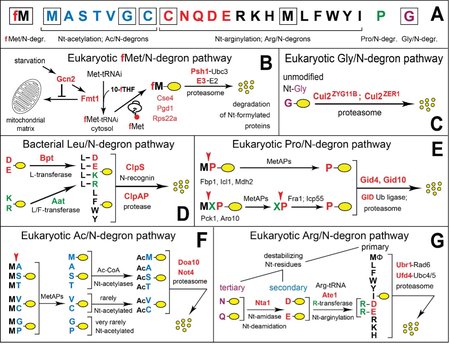
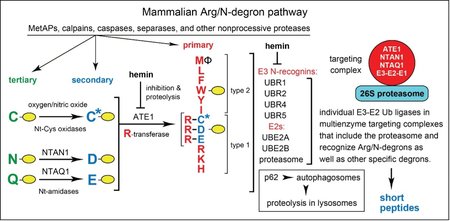
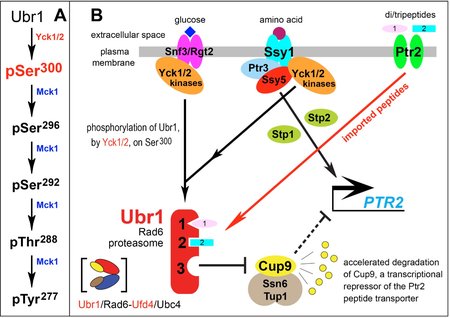
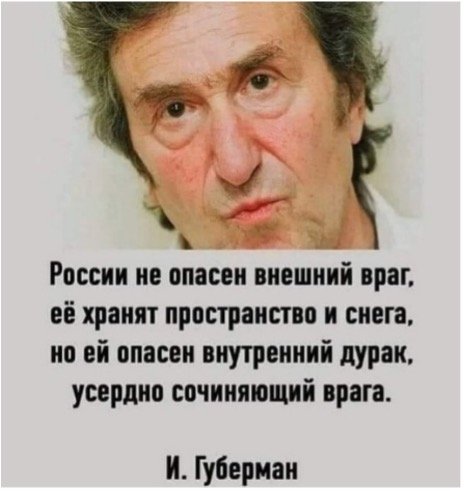
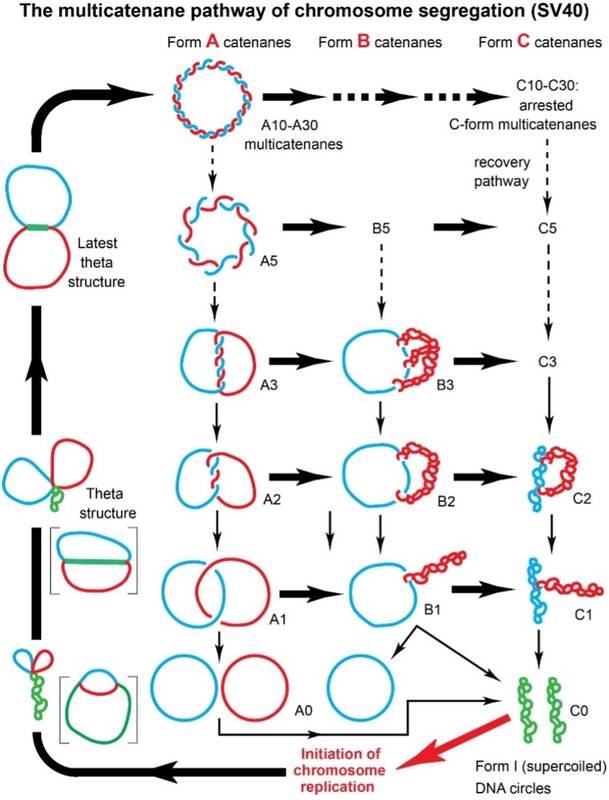
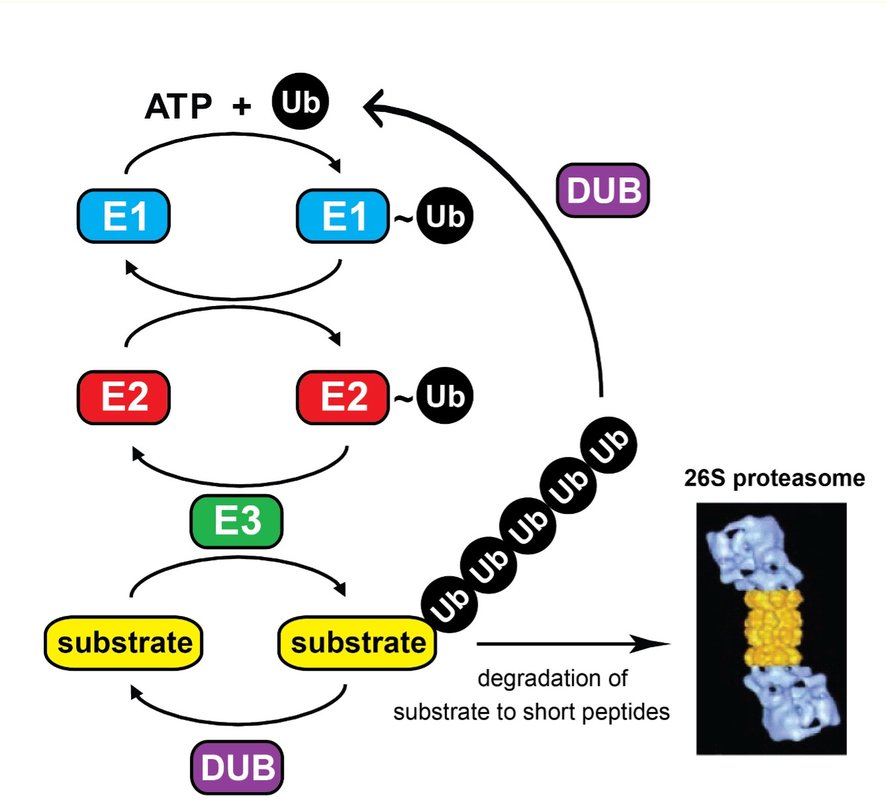
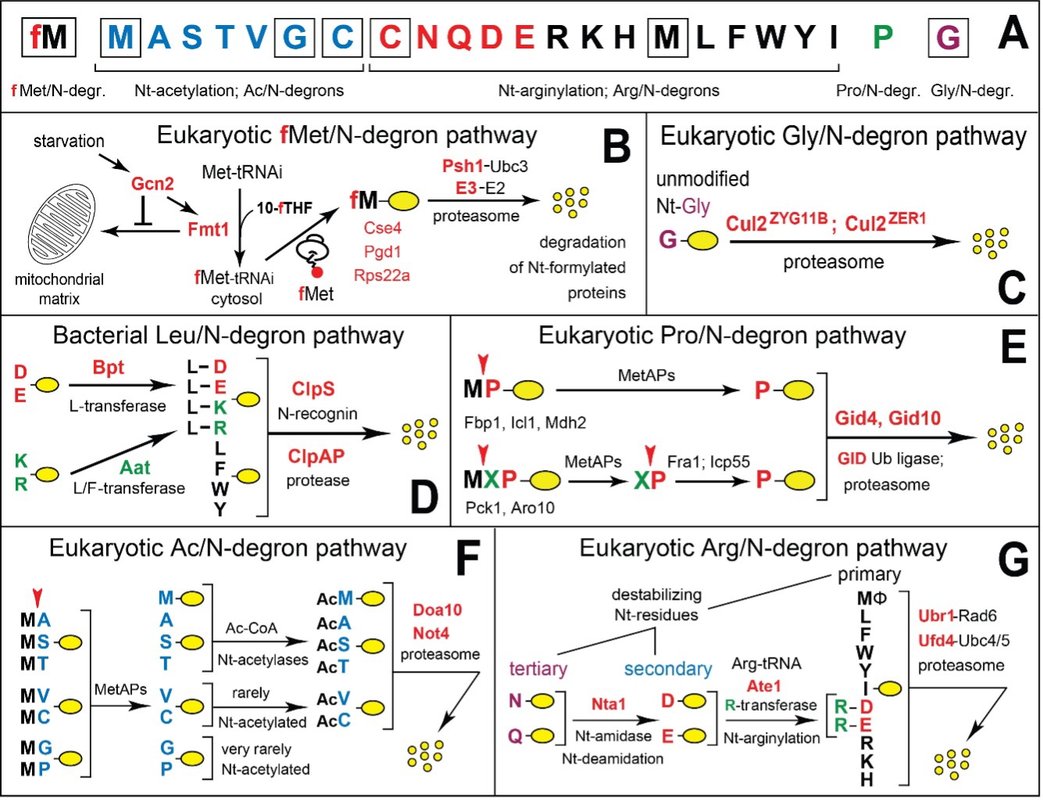
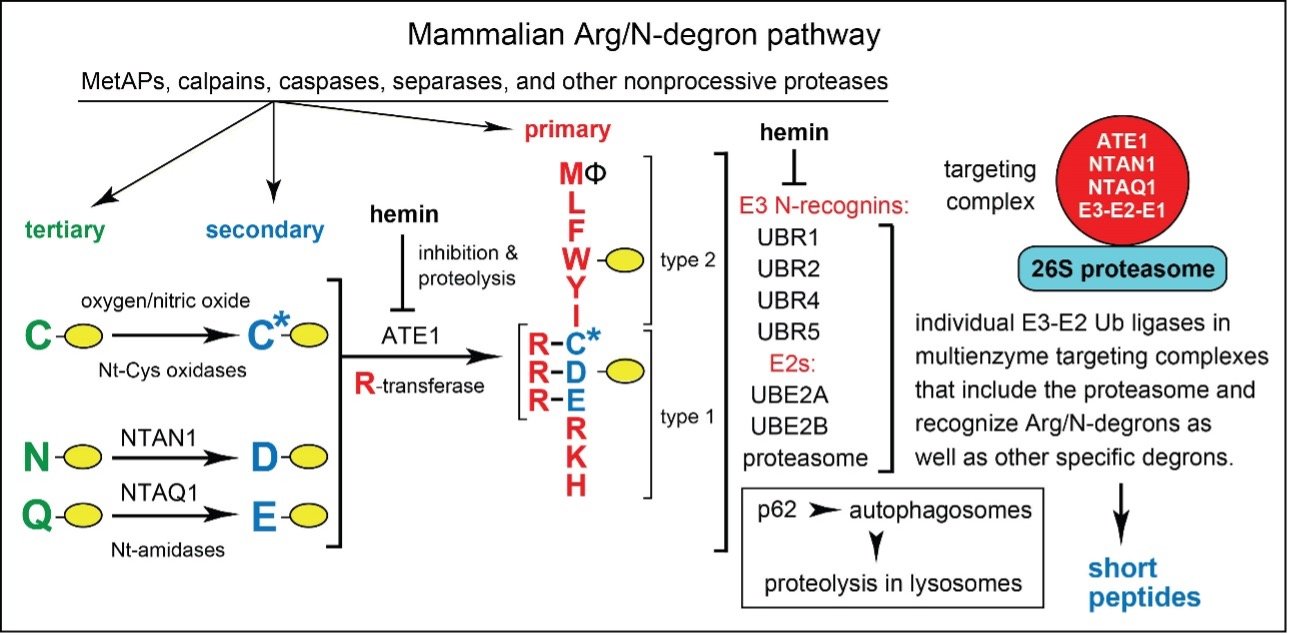
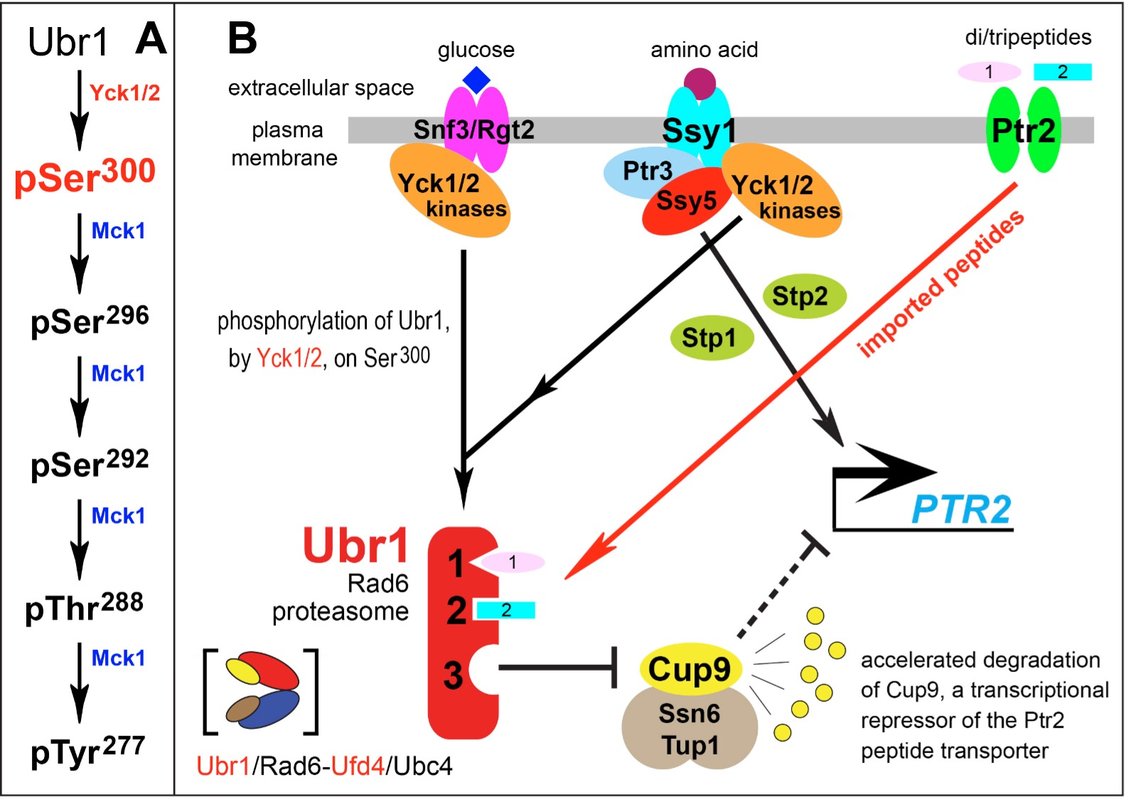