March 17, 23, and April 15, 2022
Unique among the sub-disciplines, plasma physics lends itself well to combining theory and experiment, and basic research and applications, within a single research agenda. Paul Bellan's career is testament to the broad research areas one can pursue in studying plasma, one of the four fundamental states of matter. Bellan has made important contributions to solar physics, space weather, and astrophysics, his focus on ice dusty plasmas have enhanced our understanding of Saturn's rings, and his work on fusion energy has helped get this game-changing resource ever closer to reality.
Bellan grew up in Winnipeg and became involved in plasma physics at Princeton, where he realized that a periodic antenna is a phased array which puts on a well-defined and observable wave vector. This research caught the attention of Roy Gould, and Bellan joined Caltech's faculty in 1983. Recent research includes Bellan's discovery of a new way to determine the wavelength of energy flowing through plasma in space, and he has developed a new model that explains why astrophysical jets originate from stars that have accretion disks.
Interview Transcript
DAVID ZIERLER: This is David Zierler, Director of the Caltech Heritage Project. It's Thursday, March 17th, 2022. I'm so happy to be here with Professor Paul M. Bellan. Paul, it's great to be with you. Thank you for joining me today.
PAUL M. BELLAN: Thank you. It's my pleasure to be with you.
ZIERLER: Paul, to start would you please tell me your title and divisional affiliations here at Caltech.
BELLAN: Professor of Applied Physics in the Engineering and Science Division.
ZIERLER: Why applied physics, why the title applied physics, given the fact that you have theoretical and experimental aspects of your research agenda?
BELLAN: I was hired into that. What used to be an option is now part of a department. Applied Physics was established by colleagues of mine who are a generation older than me, just a few years before I came. They felt that there was a space between engineering and physics that was missing. That engineering was trying to make gadgets or black boxes for other people to use, and physicists were trying to understand fundamental things. But, in between there was a space for people who were not quite making gadgets for consumers, but weren't only doing abstract work and they thought applied physics was that. It was this in-between space that didn't fit in the previous niches. It was copied. It was done first at Caltech and then it was copied by other institutions, so it's a bit rare but there are a number of institutions that have applied physics departments. I have changed my trajectory a little bit, in that I am doing things that are probably more fundamental than applied in many ways. But I'm kind of omnivorous and I don't restrict what I do, so some of it's applied, some of it is not applied. I do whatever. Looks like, it's a good way to use my efforts.
ZIERLER: Paul, of course, the in-between space that you talk about, there's also electrical engineering. In fact, in some institutions, electrical engineering and applied physics are used somewhat interchangeably. Where is electrical engineering for your research agenda and how it fits in at EAS?
BELLAN: I do plasma physics, and if you look at other institutions, you see the kind of work that I do, at other institutions, is sometimes in the physics department, sometimes in the electrical engineering department, sometimes in the aeronautics department. It's all over the place. Nobody thinks much about the fact that it's applied physics at Caltech and not an electrical engineering, because at some other institution it might be in another department. In some big places, plasma physics might be in more than one department. UCLA, plasma physics is both in physics and in electrical engineering. Some of it is also in more astrophysics or geophysics. It covers all those areas, so where it winds up is often a historical accident.
Plasma as the Fourth State of Matter
ZIERLER: Getting to that question, what is it about plasma physics that makes it so amenable to any number of departments and disciplines and research focus?
BELLAN: I guess the quickest answer to that is that sometimes people call plasma the fourth state of matter. If you were to, say, study liquids, that could wind up in a lot of different departments. Plasmas occur in a wide variety of areas, mainly areas that people are not familiar with a day-to-day basis. People don't have too much intuition about plasmas. But there are destro-plasmas. I could give my spiel about how important plasmas are. There are industrial plasmas. The chips in your smartphone are made using plasma processes. There is a multibillion-dollar industry there. There's a big research effort in thermonuclear fusion to make electric power. Plasma's important in the earth's magnetosphere and interplanetary space, the surface of the sun, and astrophysics. Electric arc furnaces that make steel involve plasma. There's plasma in many different areas. I think one of the interesting features about plasmas is that once you've studied it, there's a lot of commonalities in these different areas. You can jump from one area to another. I've done that. People often enter plasma physics through one of those areas. They have some problem or some interest, and they want to, say, do fusion. They want to solve the world's energy problems and they decide that fusion is the way to do it. But to do fusion you have to learn plasma physics. Then, once they learn plasma physics, they discover that what they've learned might be applicable to some other area, like solar physics. They maybe came up with something interesting in their fusion physics work that they think would be useful for solar physics. It makes them interested in solar physics. This can happen in other fields too, but it's particularly strong in plasma physics.
ZIERLER: Paul, because your research and your research group has elements of both theory and experimentation, for you, do you consider yourself really a 50-50 split, or are you primarily a theorist who does experiments, or an experimentalist who does theory?
BELLAN: I'd say, by now I consider myself a 50-50 split.
ZIERLER: Is that common? Because nowadays in physics, the field is so bifurcated, where in particle physics, in astrophysics, people are strongly one or the other. Is there something about plasma physics that makes it lendable to both sides of the equation?
BELLAN: I think plasma physics is in a more primitive state than the other fields, and it's more of a frontier area, so it's more possible to do that. Also, I think you can't really be just a plasma experimentalist. I think you really have to have pretty strong theoretical skills, even if you're a plasma experimentalist. I think it's too ill-defined and too far-ranging to just blindly do it as experiments. That's just not possible. Then, there are plenty of people who just do theory, but I'd say there are very few people who do experiments and don't know any theory. I think that's uncommon.
ZIERLER: It's interesting to consider the field relatively young, because at least the foundations of plasma physics, if I understand correctly, really goes back to the 19th Century.
BELLAN: No, I don't think so.
ZIERLER: When do you date it?
BELLAN: It didn't start all at once. Yeah, you could probably go back to Medieval times and say, this was a plasma. I think the term plasma was actually coined in the 1920s, about 100 years ago, by Langmuir. Then, there was work done in the 1920s with a certain type of vacuum tube, a voltage regulator tube that was plasma. Then, in the 1930s, there was work done on radio waves propagating the ionosphere, short-wave radio. There's a whole history of that. People didn't understand why you could send radio signals over the horizon. Marconi discovered that. There was a wonderful book called Thunderstruck by Erik Larson, I think. It describes Marconi. Marconi made the first radio and everybody was surprised it could make contact with ships that were over the horizon. People didn't understand that. Then they discovered it was bouncing off the ionosphere. A lot of theoretical work was done to understand why it bounced off the ionosphere, and that's essentially plasma waves. That was done in the 1930s.
Then in the 1940s, magnetohydrodynamics was more or less invented. I'm not totally sure of the details of that, but I guess there was some work in plasma confinement in the 1930s by Bennett, because there's something called the Bennett pinch, which dates to the 1930s, showing that magnetic forces could hold the plasma together. Then, Alfvén came up with his waves in the 1940s. Those were the beginnings of magnetohydrodynamics. Then around 1950 or so, the idea of using plasma for fusion was done, I think, independently in the US and the Soviet Union and Britain. That's roughly the history of plasmas. Then when the space age came up after Sputnik, then people realized that the whole of space is filled up with plasma and it's quite complicated. There are lots of different regions and lots of different things happening. That would have started around 1960-ish or so. The discovery of the Van Allen belts. Then probably industrial plasmas, I don't know when that would have started, probably 60s or 70s for plasma etching. I mean, you could consider welding as a kind of plasma or candle flame as a plasma, it depends on how you define a plasma.
ZIERLER: Paul, as you mentioned, it's sometimes a historical accident why plasma physicists are in one department or division than another. Is there anyone in PMA who does plasma physics, or is there a particularly obvious reason why you are not in PMA?
BELLAN: There have been people in astrophysics who are using plasmas. I think one has to make the distinction between people who use plasmas and people who do plasma physics.
ZIERLER: What is that distinction? What does that mean use and do?
BELLAN: People might be interested in something else and they have to use plasma physics to explain whatever it was they were seeing there, but they're not trying to advance the field of plasma physics. They don't have an idea in plasma physics, and they're not hunting for applications in a bunch of different fields. They have their own project, their own problem they want to solve, and they might try using a bunch of different approaches, one of which might be plasma physics. They're looking up something in a book or something to get what they want, and if they find it, then they're happy and then they move on to something else. I guess I'm not sure right now who in PMA that would be considered a plasma physicist. Sterl Phinney certainly has used plasma physics in some of his work. We used to have Roger Blandford, who moved to Stanford, but he was studying astrophysics that used plasma physics. There probably are some other people, I'm not sure. I'd have to look. I might have missed somebody.
ZIERLER: Paul, given how diverse your research group is, are there any professors or other research groups on campus that you've collaborated with over the years, in EAS or elsewhere?
BELLAN: I'm not a great collaborator at Caltech. I've collaborated more with people at other institutions. I would say I have consulted with people at Caltech, but I haven't worked closely with other people at Caltech, generally.
ZIERLER: Paul, what about JPL, has JPL been a resource for you at all?
BELLAN: A little bit. There are people at JPL who do various kinds of plasmas and there's plasma propulsion at JPL. Jay Polk is somebody who I haven't worked with directly, but one of my former students is in his group or group-affiliated with him, and I've occasionally spoken to people there. There are solar physics people that are good friends of mine. Paulett Liewer is a solar physicist at JPL, so sometimes I talk to her about what she's doing. Right now, I am collaborating with a JPL person who is not a plasma physicist, but I have something that involves ice and plasmas. He's an ice person, and we're working together on something. I have had some significant contacts with people at JPL over the years.
ZIERLER: Paul, the nice historical sketch you've provided of plasma physics from the 1920s, some institutional history questions. First, does plasma physics go all the way back at Caltech to the days of Millikan and Hale?
BELLAN: I don't think so. The person who brought me to Caltech, Roy Gould, I think was the starter of plasma physics at Caltech in the 1950s probably, maybe 1960s.
ZIERLER: What was Gould known for?
BELLAN: Oh, a lot. He just passed away recently. He did very important work on several kinds of plasma waves, on non-neutral plasmas, on tokamak turbulence. He was at the Department of Energy and he was the one who authorized the building of the largest tokamak in the United States when he was at DoE. He did important work in microwaves. He was a microwave person who moved into plasma physics. He was one of the people who started the applied physics option, and now department.
ZIERLER: Paul, let's go to the theoretical side. Over the course of your career, what have been some of the most important theories in physics that serve as a guidepost for your research?
BELLAN: Theories in physics? Plasma physics is largely classical. There's not a whole lot of quantum mechanics in it, although there are limits where—peripheral areas where quantum mechanics comes in. I remember going to a talk where somebody was scribbling on the blackboard all of the things that could go into plasma physics, and he drew 20 different areas of physics, with arrows all going in, and he said, "plasma physics is like a black hole for physics, everything goes in, but, some people claim, nothing comes out." So there's an awful lot of ideas in physics that go into plasma physics. Basic plasma physics involves electromagnetic theory, Maxwell's equations, and particle motion, the Lorentz force, because if you have a charged particle, it gets pushed around by electromagnetic fields. Then, Maxwell's equations tell you how those fields evolve, how currents and charged density make those fields. But then, if you have charged particles moving around, then the charged particles make electric fields and make magnetic fields. That's what plasma physics is, it's the self-consistent motion of charged particles that are acted on and by electric and magnetic fields, and that also make electric and magnetic fields.
Then there's a many-body problem aspect to it. When you study physics, you learn about the two-body problem, about the earth orbiting the sun, and then your professor tells you that the many-body problem is impossible, and nobody's ever figured it out. Plasma physics is the many-body problem, where you've got huge numbers of particles and you have to kind of figure out how to deal with that. It has elements of statistical mechanics in it. It's related to wave theory a lot. Plasmas are very rich in waves. There are all kinds of waves, from acoustic-like waves to electromagnetic-type waves to waves like hydrodynamic waves. Any kind of wave you can think of, there's some variation of it in plasmas, and then there are waves in plasmas that don't exist at all, even conceptually, in other fields.
There's non-intuitive aspects of plasmas. That is, in most other physics, you're taught that things always go a certain way and, say, if you make something hotter, it gets more resistive. Physicists are always cooling things down to really cold temperatures to make them work better, and plasmas are the opposite. If you heat things up, then there's less friction and less resistance, so your intuition of that is wrong. Plasmas have some connections to superconductivity. In one limit plasmas behave like a superconducting gas. Ideas of what it means to be a superconductor come up. There's things related to black-body radiation. Many different kinds of physics get involved in plasmas, and so I think that's one of the things that makes it fun. It has a great range of parameters too. If you, say, take solid-state physics, you're dealing with matter and crystals and the atoms are separated by a certain distance that's a few angstroms and the temperatures, room temperature, or maybe a little bit colder, a little bit hotter, and the density is always about the same, so you're in that regime. Whereas plasmas, the numbers can be all over the place. The density could be one particle per cubic centimeter or could be ten to the power thirty particles per cubic centimeter. The dimensions of it might be microns or it might be a million light years. The neat thing is that the same concepts apply, to a large extent, in all these different areas. That's why you can jump around to all these different areas.
ZIERLER: The big takeaway here, obviously, is that plasma physics really requires you to be a generalist in the field.
BELLAN: Yes.
ZIERLER: Paul, on the experimental side, over the years, either thinking about computation or technological advances or material science, what have been some of the advances that have served as game-changers, in terms of making experiments possible that weren't before?
BELLAN: I've been around long enough to see some of that. These advances have come from outside of plasma physics, and I think they're not unique to plasma physics, I think every field has enjoyed the same thing. The fact that electronics has become much cheaper, computers much cheaper, of course has made a big difference. I think the idea of going to multiple channels of measurement is a big deal. It used to be, you'd have a plasma and you'd put a probe in, and you'd measure things at one position. You'd get maybe a time history on an oscilloscope, and you'd take a picture of the oscilloscope with a Polaroid film, and then with a ruler measure the Polaroid film. That's the way things were when I started. Now you can have a thousand channels or ten thousand channels, or a hundred channels, depends on what it is. But many, many channels. Of course, you can record all this in a computer, and a computer can analyze it, so the amount of data you can collect is orders of magnitude more than before.
Then, of course, you can design experiments much more cheaply. It used to be, you'd sketch something up, and you'd have to make a drawing by hand or get a draughtsman to make it, and take it to a machine shop or do it yourself. Now you can draw something in a 3D CAD program in your computer at home, in your office, and then email it to some company, and give them your credit card, and they'll mail back a complicated 3D part that's relatively cheap. That allows you to do a lot of things that you couldn't have done before. Then, of course, electronic parts are very cheap. They're practically free compared to what I remember, so there's a lot of possibilities for building things. You're not really limited so much by stuff anymore. It's more you're limited by time and money and ability to support people, but the actual hardware, I'd say, is fairly accessible.
I think a feature about experimental plasma physics compared to other fields, is that it's a small field and there's not a whole lot of catalog equipment that you can buy. In some other fields, if you, say, have a beginning assistant professor, if they're given startup money and they can just buy several hundred thousand dollars' worth of stuff out of catalogs, and then they're off and running. You can't do that in plasma physics. You, essentially, have to build everything yourself. You can buy some instruments, but your own specific stuff, you have to build yourself, so the fact that it's easier to build things now I think makes a difference.
ZIERLER: Paul, has your work relied on computer simulation over the years?
BELLAN: I wouldn't say it's relied on it. I've always done a little bit of numerical work, but I was never an expert at it. I was just very much an amateur. For a while, I resisted getting too involved in it. I did some more serious things about 30 years ago, and once you start doing numerical work, it's like a black hole for time. Another kind of black hole. If you've ever done anything with computers, you can sit all day long and then wonder where'd the time go. Your wife is calling you for dinner and you thought you'd just started. I felt that level of numerical work should be left to the people who want to do it full-time, but I've dabbled in it a little bit, and have collaborated with people who do numerical work. I encourage my students to learn how to do numerics at a modest level, so that they're aware of what can be done. I think it's very useful if you're doing things that are not too big. If you can do something in a day or two, or an afternoon, numerically, then I think it's a good use of time. What you can do that way has, of course, changed over the years, as computers and techniques have changed. But I'm not in favor of spending a whole year just doing that and nothing else.
We have done numerical simulations more and more in recent years. I collaborated with Hui Li at Los Alamos a few years ago, who had a massive three-dimensional MHD code, and I sent students there to learn how to use it. We used that to simulate one of our experiments in quite a bit of detail and had students doing quite a bit of simulation with that. It depends on the student. Another student used the publicly available particle-in-cell code to do quite a bit of very impressive work. I guess I have supervised students who put the time into it. I haven't done it too much myself. I had a graduate student about 20 years ago who was a real pro at that and who was co-advised by me and Dan Meiron in applied mathematics and she did a superb thesis that was all simulation. I'm somewhat involved in that, but I try to be careful about that. I know there are people who are much better at it than I am.
ZIERLER: Paul, an overall question, as it relates to your motivations in science. Because some aspects of your research are fundamental, and some are really applied, when do you make the decision about whether to figure out how something in nature works, and when to focus on your efforts an application that might be beneficial for society?
BELLAN: My late father was an economist, and I was brought up with dinner conversations about economics a lot. I think I've been biased a little bit by that thinking, so I feel that I make decisions based on where I think my effort is best used. If something comes along and I think that, hey, I can do something there, then I will do it. It doesn't really matter whether it's pure or applied. It's just, I think, there's a sort of profit to be made, I can make some progress there. But I don't believe in hitting my head against the wall trying to do something that is not working out very well, because that was the goal and I have to stick to my goal. I mean, I've changed a little bit over the years in that regard. I even wrote something in which I spoke about the importance of being opportunistic, and I've looked at historical analogues of that. Like Columbus set sail to go to India and didn't make it. He was a failure, didn't make it to India, but he found America instead, and people decided to take advantage of that. They didn't say Columbus, you're an idiot, you failed. They were kind of happy that he discovered something. I think you should, at any point in time, you're working on something and there's something else that looks like an opportunity, you have to always decide, should you finish up what you're working on now or should you start on this new thing that's coming up. You have to make some kind of estimation, what the return will be on your effort, and you try to pick the thing where you're going to have the most return on your effort. It doesn't have to be a practical return, it could be a theoretical return, or it could be fundamental physics or abstract or whatever, but it should be a return according to how you feel, in the sense that it makes some kind of impact or progress.
ZIERLER: Paul, staying on the fundamental side, in surveying your contributions, how has your work and the work of your colleagues more generally in plasma physics, moved the ball forward in some of the fundamental questions in physics?
BELLAN: I think that's hard for me to say. It's hard to gauge that. Partly because this is a field where everybody makes some little contribution, and the progress is kind of an accumulation of all these little contributions. I don't think I can claim that I made some spectacular contribution that changed the field around, but I know people are using some of the things that I've done.
ZIERLER: If I can pull you out of your modesty a little bit, what are people using? Or what are people advancing based on your contributions?
BELLAN: I don't know. I'd rather flip it around the other way, is that there are plenty of things that I did, I've done, where I think people should be using, but I think they're not, because they haven't figured it out yet. The field is one where, there's a lot of complexity in the field and so I work on something, but there's just a few other people working in the same area. It's not as if I do something and then hundreds of people are now working on it. I'll do something and maybe a few other people will pick up on it. I don't know, I have to think about it. One of the things I feel with a lot of the stuff I've done, is that it's a little bit hard to explain to people what it is, because it usually involves understanding things in a different way from how people understood things before. That's an issue. I guess something's starting to gel in my mind to answer your question. It's not obvious. One of the things that I worked on, just as I started in the 1980s or so, was something we called stochastic ion heating. I think that has become fairly important in a number of areas. I'll have to give you a brief lecture on plasma physics, to put that in context.
ZIERLER: Please.
BELLAN: In plasma physics, you have magnetic fields, and this picture is, you've got a uniform magnetic field as the simplest model. Say, straight field lines in the horizontal direction, and then there might be an electric field also, and for now, restrict consideration to electric fields that are perpendicular to the magnetic field. We can also talk about parallel component, but that's something else. When you have electric and magnetic fields that are perpendicular to each other, there's various motions that occur. If you have the magnetic field only, the particles execute what are called cyclotron or Larmor orbits. They go in circles around the magnetic field. I guess I don't know how much physics background you have, so maybe—
ZIERLER: Enough, enough. I'm tracking with you.
BELLAN: —you're tracking, first, particles make cyclotron or Larmor orbits around the magnetic field. Then, if there's an electric field, they also have a drift superimposed on that, so they will move in a direction that's orthogonal to both the electric and the magnetic field. Then, if the magnetic field is non-uniform, if it has gradients or curvature, there's other drifts, and if there's gravity, other drifts. There's a whole theory based on that called guiding-center theory, where there's a hierarchy of motions, of Larmor orbits, and what are called E-cross-B drifts, and curvature drifts, and grad-B drifts.
Then there's also a concept called adiabatic invariance, which says that when particles make these drifts, there's something that's conserved. It's somewhat analogous to a quantum number actually, when you look at it. It's something that comes out of classical mechanics. In particular, the simplest one has the temperature of the particle perpendicular to the magnetic field direction, divided by the magnetic field, to be a constant, even if the magnetic field is changing or curved or anything's happening, that ratio stays constant. What we found is that if you have a wave that is moderately strong it can destroy all this. This idea that the particle makes Larmor orbits and the drifts, all falls apart. The particle starts to develop a chaotic kind of motion. There's no longer this adiabatic invariance, that the temperature or the magnetic field is constant, so everything kind of falls apart. This is analogous, if you've ever done any numerical work, of trying to solve a differential equation and picking a step size that's too big, and your code goes to hell because things jump all over the place. That is what happens with the particle. It's motion becomes very erratic and chaotic.
We saw this experimentally first. I had a little tokamak in those days, and we set up a diagnostic to measure the ion temperature. We were assuming the ions would be cold, because in a plasma like that, ions are always cold. Much to our surprise, the ions were not cold, they were much hotter than we expected, but only in a certain region of the edge of the plasma. We couldn't understand that, except there was a wave, called a drift wave, at the edge. The drift wave was very coherent, and according to wave theory, if waves are coherent then they shouldn't do much. Maybe if there is turbulence, they should do something. But we had this very clean drift wave there, and in the meantime the ions were getting very hot. We couldn't understand that. Then we discovered that this drift wave was causing this chaotic motion of the ions. It's philosophically rather interesting, because it's not a true heating, in the sense of a frictional heating or collisional heating, it's actually a deterministic sort of heating. That is, you could still actually follow the trajectory of every single particle. There's no increase in entropy, strictly speaking. But the particle trajectories are very sensitive to initial conditions, just like the butterfly effect that you've probably heard of.
So you can duplicate this in a computer. You set up a bunch of particles in a wave such as a drift wave, give the initial conditions, and if the drift wave amplitude is below a threshold, then the particles just move along in a nice coherent way. But if the drift wave amplitude goes above a threshold, then the particles go haywire, and you get this heating. This is now, I think, a reasonably well-established concept. Other people also thought of this around the same time, but I think we were the first ones to really see it experimentally and to make the identification that this is a real effect. I think we did some of the good theoretical explanations of it. More recently, we did some work showing that this is likely going on in magnetic reconnection, which is a contemporary hot topic in plasma physics.
ZIERLER: Why is it a hot topic these days?
BELLAN: Plasmas with magnetic fields are an important part of plasma physics, so fusion plasmas, solar plasmas, astrophysical plasmas, earth's magnetosphere, all have magnetic fields. As I said, when a plasma is very hot, it becomes like as if it has no friction, and then it becomes like a superconductor. The essence of a superconductor is that the magnetic flux is frozen in to the medium. Plasmas are like that, to the extent that they're hot, magnetic flux is frozen in. You can think of the magnetic field lines roughly as being frozen into the plasma. If that were really true then the plasma topology could never change, it would be stuck in the same topology because the lines could never move across the plasma. I have a somewhat grotesque analogue for that. When a baby is born, the umbilical cord is cut. But imagine some other planet where the umbilical cords are never cut, imagine what animals and people would look like. To get discreet objects, you have to cut things. But if you have magnetic structures or plasmas frozen in magnetic field and you could never break off the magnetic field, then nothing would ever get unstuck. It would be like this world where you never cut the umbilical cord.
So it turns out that magnetic fields are mainly frozen in, but they do get cut now and then. It's a rare event, but that's how you get things separating, otherwise you never get anything separating. The cutting is somewhat of a dramatic thing. All sorts of sparks fly, effectively. Another analogue, which I've actually used in TV interviews, is blowing soap bubbles. If you blow a soap bubble, it's on the wand and gets bigger and bigger, but then it breaks off. If it never broke off, then it would just stay there. Magnetic reconnection is like this process of breaking things off. If I asked you to explain how a soap bubble breaks off, you'd say, well, that's a difficult problem, there's obviously a lot of things going on. You have to worry about this and that and the other thing. Magnetic reconnection is same deal, there's a lot of complicated things going on. You get complex effects with large electric fields and you get particles accelerated to high-energy and you get all sorts of waves being generated. If you go back to the bubble analogue, when you blow off a bubble, you hear a pop. Then you can say, well, explain the pop. You say, then you have to learn about waves and how you generate a wave. Why does it pop? It's because the surface tension can't do this and the pressure's too much. Magnetic reconnection is full of stuff like that.
Anyways, the stochastic ion heating we argued was something that's going on in magnetic reconnection, and you get some reasonably dramatic stuff happening there. People have often seen extreme ion heating to temperatures much higher than the electrons when there's magnetic reconnection. Often the electrons are hotter than the ions, so you'd expect to find they'd be heated by the electrons, but it's pretty hard to explain that if the ions get hotter than the electrons. The question is, how do the ions get heated up. We proposed an explanation for that, and that was demonstrated by simulations. That maybe answers multiple questions in one shot.
The Prospects for Fusion Energy
ZIERLER: Paul, some questions on applications. Of course, there's always been excitement about fusion energy. The joke is, that it's been just around the corner for the past 40 years. Before we get to where fusion energy is now, what have been some of your contributions to making fusion energy more of a reality?
BELLAN: My earliest work, from when I was a graduate student, was fairly closely related to fusion. I studied a particular wave. Again, there are many kinds of waves and plasmas. This particular one was called a lower-hybrid wave, and it was proposed as a means for heating tokomak fusion reactors, and later, as a means for driving the current in a tokomak fusion reactor. I think it's still one of the candidates for that. There are other things that are getting more prominence now, but it's still under consideration. I did some of the very earliest work on how lower-hybrid waves propagate. They propagate in a way that was literally orthogonal to intuition. If you study waves, there's a dispersion relation, that is an equation that characterizes waves. When you study dispersion relations, it talks about the frequency and the wave length and the wave number and it has a direction associated with it. It turns out that that's, I don't want to say wrong, but it's sort of misleading for describing what's going on. That the direction that the dispersion relation gives you, is actually perpendicular to the direction that the energy goes in. Maybe if, have you heard about group velocity?
ZIERLER: Sure.
BELLAN: When you study group velocity, you learn that group velocity is different from phase velocity. These waves have a group velocity that is perpendicular to the phase velocity, so that definitely throws a spanner in the works of your intuition. I always found that very interesting and have built up a good intuition of how that works. Did a fair amount of work on how to get lower-hybrid waves propagating into the tokomak. This was a long time ago. I haven't really worked on that lately. But that was one of my fusion contributions. I'm trying to think whether other things I've done have had a whole lot to do with fusion. I've done some work on drift waves, which have some peripheral connection to fusion.
Also, I've done a lot of work in spheromaks, and spheromaks are a proposed fusion device. I guess from that point of view, that was pretty closely related to fusion. Spheromaks were touted as the smarter, cheaper, faster alternative to tokamaks, and smaller. They came on the scene I guess in the 1980s, and they were very intriguing. The picture I have for that—sort of my standard spiel is that, you go to a junkyard and there's all these rusty auto parts sitting on the ground, covered in dirt, and you throw them up in the air, and you throw them up real high, and they collide with each other, and they happen to collide in such a way that they piece themselves together to form a brand-new BMW that slowly wafts down. Then you drive off in this new BMW, so that's what we would call self-organization, that you'd get. Spheromaks have that element to them. It basically works, the only problem is that what comes down isn't a brand-new BMW, but it's a rusty old Volkswagen, but it still drives. It leaks oil, but there is self-organization, it just doesn't give you quite as nice a thing as you'd like. Then you ask yourself, well, can you do something with that? You got it for free more or less, and it's better than nothing, and maybe you can still make fusion that way. There's a bunch of people working in that area, and I guess I'm collaborating on something relating to that right now.
The sort of big question then is, how does the self-organization work? It sounds almost like a religious kind of argument. How could an organism as beautiful as the eye develop from nothing? It must have been something that a divine entity crafted. Here we've got something that is got some organization, that appears out of nowhere, and how's that work? There are theories for that, which intrigued me, and I've done a lot of work on that. I think it has all sorts of interesting philosophical implications. Much of the work I've done in the last 20 years or so was, at least originally, motivated by trying to answer that question. How does the self-organization work?
ZIERLER: Paul, I'm intrigued, what do you find that raises philosophical questions about this?
BELLAN: Yeah, how do you get something organized out of nothing? If you start with the universe being a pile of randomly moving gases, how do you wind up with organized anything? There is a lot of organization, and so why should there be organization? I'll tell you the spheromak argument, and I think that there must be analogous arguments in other contexts, they'll be different players in the game, but it'll be a similar one. If you know physics, you know about entropy and you know about energy and you know about maximizing things and variational arguments and about constraints, so you can set up a model of something or other where there's a few of these things involved. Say, in thermal or in statistical mechanics, you deal with something like entropy and energy, and you would say that if you make a system, you put it in a box, you let it evolve, it will try to go to the state of maximum entropy, but energy is a conserved quantity, so it will have to maximize entropy while conserving the energy. Then you put that in an equation form and use your variational calculus and you'll wind up with Maxwellian velocity distributions. That's what you get when you do that.
But then you could imagine some other situation, where instead of entropy and energy, you've got another pair of things where you're maximizing something while constraining something else. In magnetohydrodynamics, there's two concepts. One is magnetic energy, which is the energy that is contained in the magnetic fields, that's of the volume integral of B squaredin a magnetic field. The other is a very intriguing concept called magnetic helicity, which is a measure of the linkages of magnetic fields. If I put my fingers together and I make a linkage like that, you can imagine magnetic fields being linked like links on a chain. You could imagine a box full of magnetic fields and there are lots of little linkages, like that. If you could figure out some way of counting up the linkages, then you made some number that told you you've got a thousand linkages or so, that would be the helicity. It turns out that counting up the linkages is not difficult to do mathematically. You've heard of vector potential?
ZIERLER: No, what is that?
BELLAN: You've heard of magnetic field?
ZIERLER: Yeah.
BELLAN: You've heard of the curl operator?
ZIERLER: Mm-hmm.
BELLAN: If you think of electric field, the electric field is the gradient of a potential, or minus a gradient of a potential, if you have electrostatic potential. You can write the magnetic field as the curl of something, which you call capital A, and that capital A is called the vector potential. Just like the electric field comes from an electrostatic potential, you could have a magnetic field coming from the curl of something. This vector potential is a screwy sort of thing, because it's a bit ill-defined. You can add a constant to it, or actually you can add more than a constant. You can add an almost arbitrary function, that's a gradient of a potential, and it doesn't change the magnetic field. It's sort of non-intuitive and it's not only non-intuitive, you can add things to it to make it look different, and it doesn't make any difference. It's a bizarre thing. Anyways, it turns out that the way to count the linkages is you take the magnetic field and you do a dot product. You know what a dot product is?
ZIERLER: Yeah.
BELLAN: You do a dot product with the magnetic fields, you have A dot B, and you integrate that over to the volume of interest, and that will count up all the linkages. It's not a hard thing to do. There was a very influential idea, I guess it was done first in the 1950s, and then sort of forgotten, and then done again in the 1980s by a different person. Where they claimed that a system, a magnetohydrodynamic system, would evolve in such a way that the energy would minimize. This is different from statistical mechanics, where we're conserving energy. Now we're not conserving the energy. We would try to go to the lowest energy state, but it would have to conserve the helicity. If it started with a thousand linkages, it would keep a thousand linkages. The linkages might unlink, but every time they'd unlink, they'd make another linkage in different ways, so there would still be a thousand linkages.
You can explain why this is the case. The explanation is a strange explanation, because most ideas in physics sort of work, but they only work when the system is very clean. If things start getting dirty and messy, then they don't work so well. You have to make the system very pristine, and it works. But this argument turned out to work better when the system got dirtier. As you made things more and more messy, then the argument gets better and better. Which is an interesting idea. If you have a really messy system, and you set it up and let it be turbulent and have horrible things going on that you can't understand, and you wait a long time, you can show that it will tend to a state where it has a minimum energy, which can't be zero, because then that would affect the helicity. It has minimum energy while conserving the helicity. When you solve that, you get some relatively simple equations. Those give you a state and a spheromak is that state. That's where the self-organization comes from. You make a box and you put plasma in it and it's as turbulent as all get-out and you don't even attempt to follow what's going on. You just wait, and it'll settle down to this nice state. This spheromak is a very close cousin of a tokamak, it has similar magnetic geometry. It's basically the same thing as a tokamak, but without a hole in the middle. If you look at tokamaks, if you're familiar with them at all. The main thing you see is they're like donuts with a hole in the middle. If you fill in the hole, then you've got a spheromak.
The claim is that you just get a box, stick in a plasma, let it do what it wants, and it'll settle down to a spheromak, and now you've got a tokamak-like thing for free. This seemed pretty bizarre when I first heard about it, and other people thought it was pretty neat too. Several groups tried to make spheromaks and used very different approaches. They said, "oh, my method will work, yours is no good, you don't know what you're doing." What happened is that they all worked. That's because, nature really wanted to make spheromaks. It didn't matter, as long as you put in the right ingredients, it would just do it.
ZIERLER: Paul, given you've been around fusion for so long, how do you understand the perennial hype, that we're always just around the corner? And are things going to change at some point, are we really going to see a breakthrough in the near-term?
BELLAN: I don't think there's hype. I mean, there has been tremendous advances in fusion. I know how it was when I started. It's like the difference from the first spacecraft to nearly landing on the moon now, that's the situation we're in. There's been enormous improvement. I guess the current situation is that the ITER tokamak, are you familiar with the ITER?
ZIERLER: Yeah, sure.
BELLAN: That's an enormous machine. It costs 20, 30 billion dollars. It took about 10 or 15 years to design, and it's been under construction for about 20 years or so. It's a very conservative design from the plasma physics point of view, so I'd be extremely surprised if it doesn't work. I think the issue there is more the engineering, that something might break, and the burnout of a coil or wall might melt or something like that, and then they'll have a long time fixing it, or people will say it's never going to work because of that. But the physics part, I think, it's relatively modest change from existing machines. With tokamaks, the big deal is that bigger is better, and it's a square-cube law thing, where the losses go like the surface area and the confinement goes like the volume. If you make something bigger, it works better. The main thing about ITER is that it's bigger by a factor of, I don't know, 10 in volume or so, than the biggest machines now. Cost is a factor of 10 bigger, also, so that's it. It's designed with a safety margin of a factor of a few, so I think it probably will make fusion. I mean, the current large tokamaks are just on the verge of making fusion. The JET tokamak in the UK was within about a factor of 3, of break even, so to go beyond that isn't a big extrapolation.
I think tokamaks will make fusion. The issue really isn't whether they'll make it or not, but whether it'll be economical. I think, even there, it's not so bad. I had my students, some years ago, go through ITER and try to figure out how economical it'll be. It's not meant to be a commercial thing. It's not going to work reliably. It'll work for an hour and then break for a month. But, you could imagine that it did work reliably and it was on continuously, and then you could say, well, let's say it costs 20 billion dollars and it puts up this amount of electricity, how does that compare with existing power plants. It's not competitive, but it's not off by a ridiculous amount. It's maybe a factor of 10 higher or so. You can say, well, that's the first one, they'll be improvements and maybe other energy will become more expensive. It's not crazy. That's ITER.
Then, there's been a host of competitors to tokamaks, because everybody's reaction right away is that this is so big and so expensive and it's taking so long, can't you do it in a smaller, faster, cheaper way. There has been a lot of interest the last 40 years in that, and spheromaks were one of those, and then there are some other things that are similar to spheromaks. People worked on those, maybe two or three, devices of that ilk. The last, I don't know, 10 or 20 years, people have started raising money from venture capitalists for these smaller machines. In general, they have performance that's nowhere near where tokamaks have. I mean, it's a joke compared to tokamak performance.
But people feel the proper metric is to compare them to tokamaks that cost the same amount of money or that have had the same amount of effort put into them, and then that metric, then they're sort of interesting. Because if you have a device that somebody's worked on for 10 years and maybe 50 million dollars has been put into it over those 10 years, you can't really compare it to a machine that's been worked on for 40 years and that 20 billion dollars has gone into it. It's not a fair comparison. But if it's sort of comparable to the way tokamaks were, when a similar effort had been put into them, or maybe better than tokamaks at that time, then you're encouraged to keep on working on it. There are several private companies, at least five or six private companies, with various amounts of funding working on these. One of them, that's connected to MIT, is—well, it's a tokamak, but it involves much higher fields.
ZIERLER: This is Commonwealth Energy you're talking about?
BELLAN: Yes, right. That looks pretty serious to me. They've raised nearly two billion dollars. But it's pretty classic tokamak stuff there. They're pushing the strength of materials, essentially, by going to high fields. It's interesting, the magnetic field in a tokamak doesn't really do anything. It's like the rebar in concrete, it prevents the plasma from going unstable. If you make the magnetic field—at least this is the magnetic field from the toroidal field coils. There's also magnetic field from the current. The magnetic field is pointing in two different directions. One direction is like the rebar, the other direction provides confinement. If you can imagine if you had concrete and you could put in rebar that was 10 times as strong, and then you could have a concrete structure that you stressed much more. That's what the Commonwealth approach is. MIT has a long history of working with high field tokamaks. But it is a question of strength of materials and being clever there.
Then, there are devices called FRCs, which are a little like spheromaks, but actually simpler than spheromaks, and Tri Alpha Energy has that. There's a company in Canada General Fusion that's kind of vacillating between spheromaks and FRCs and tokamaks. There's the University of Washington, Zap Energy, that I have some slight connections with and possibly collaborating with them. I don't know whether I will or not, but at least I'm familiar with what they're doing. There's a whole range of things that people are trying, but there seems to be some private money available for all these things right now, which wasn't the case in the past.
ZIERLER: Paul, what have been some of the connecting points in your research between fusion applications and astrophysical jets specifically, and astrophysics generally?
BELLAN: That's got a really good answer. We go back to spheromaks and the self-organization, that was explained in terms of minimizing energy while conserving helicity. That's a nice thing you can write on a piece of paper. But then when you make a spheromak, you can say, well, what actually happens, what's the dynamics? What does it actually do when it self-organizes? I posed that question to myself and decided to make an experiment where I'd force the plasma to reveal what it was actually doing. How was the baby born? We designed an experiment where instead of trying to push parameters, trying to get better performance, better temperature or confinement time or whatever it is people are trying to do, I wanted it to be optimized so you could understand what was going on. I designed it to have very good access and to allow movies of what was happening. We started seeing very interesting things, and my then postdoc and I realized that this was somewhat connected to astrophysical jets. I hadn't thought of that when I was building it, but I effectively had made a model astrophysical jet. It became a dual-purpose experiment, depending on who was funding it. It was a spheromak experiment for fusion, or an astrophysical jet experiment for simulating astrophysics, and it was basically the same experiment, it was doing both. I've been really doing that for the last 20 years. It has the same initial conditions. It starts off being a jet, the jet goes unstable, and in certain regimes the instability causes the jet to break off and form a spheromak. We're still studying that.
ZIERLER: Your work on Saturn's rings, was that more of a one-off, or do you have a larger planetary science component to your research?
BELLAN: I'm getting into that. The Saturn ring thing was kind of a dead end at the time. I never really published anything on that. I spent a lot of effort looking into that, and read up a lot on it. I might get back to it, because I'm doing stuff now that is kind of related to that now. I'm more interested now in protoplanetary disks, which would be the ring around a star, but it's similar kind of physics.
ZIERLER: Stars can have rings around them?
BELLAN: Yes. It's essentially one ring. Actually they can have multiple rings. Have you ever seen pictures from the ALMA Radio Telescope in Chile?
ZIERLER: Yes, yes.
BELLAN: Some of those pictures show multiple rings. It's probably somewhat related. But I've put together a theory a few years ago, trying to relate the protoplanetary disk from the accretion disk around a star with the jets and try to describe it as one integrated system. I'm actively working on that sort of thing right now.
ZIERLER: Is your work on rings around stars broadly related to your contributions in solar physics, would you say?
BELLAN: Well, it's a little distant from that. The thing is, the solar physics—the answer is yes and no. In the sun, you see these arch-structures, and then the other thing we were talking about was astrophysical jets, and according to my way of looking at things, and I can't say that I've convinced the whole world of this, but I'm pretty sure I am right, solar loops are somewhat equivalent to two astrophysical jets that are pointing at each other. It's as if you have a jet here and a jet here and they're going up and they're smashing into each other. I mean, you make that in a lab, we can see all that going on and we have numerical simulations. In that sense, there's a relationship. It's more a question of the shape of things, but the physics is governed by the same equations, so the astrophysical jets have coaxial symmetry.
Now the question is, how do you power the jet, why is there a jet, and what's driving. These jets, observationally, are always associated with accretion disks, which is like a big version of Saturn's ring. You think of the whole solar system, of the planets, going out to Pluto or whatever. Before there were planets, there was a disk of gas extending over that area, spinning around, and then eventually some of the gas coalesced and formed the planets, and then a lot of the gas was lost. This is sort of an early stage of star formation. Before there were planets, maybe as the star was forming or just after it was formed, there was a period where there were these disks and there would typically be jets associated with the disk. People in astrophysics tended to either study jets or study disks, but they never really tried to understand why they were associated with each other, except observationally they seemed to be related. I mean, observationally they'd see that there was some connection between them. That the amount of stuff being shot out of the disk was proportional to the amount of stuff accreting. The amount of stuff being shot out of the jet was related to the amount of stuff accreting in the disk, but the actual dynamics of how the jet was being driven and what the power supplied for it was, was never really addressed. I thought our experiment provided a lot of insight into how that might work, because in our experiment we have a power supply driving our jets, so you have some sense of what kind of power supply you need, and the question is, what's the astrophysical equivalent of that. I've been working, I don't know, I think since 2008 or so, kicking out ideas now and then on how you power the jet. We're continuing to work on that right now. For that, you need to understand the disks, and then I also have this work on dusty plasmas that came about for rather different reasons, but it turns out that that is related to disks also. It's sort of fortuitous that I have some ideas for disks both from the connection to the jets and also from my dusty plasma work.
ZIERLER: Paul, for the last part of our conversation today, some questions that focus on your role as a professor and mentor to students. First, on the graduate and postdoc side, because your research obviously has elements of both fundamental science and application, how does that translate into the kinds of careers that students in your research group have pursued? Albeit in government, in academia, and in industry.
BELLAN: I guess, they've done all of those things. I guess about five students or so have become professors, doing things somewhat related to plasma physics. Then, some have gone to national labs, this is more postdocs. Some have left the field. One went to work in geophysics in the petroleum industry, with equations that are somewhat similar to plasma equations. I think he's now working at Google. Some have gone into national security, working on things I don't know what they are, but they're probably related to radar or things of that sort. Some have gone into medical device research. One of them was working at a company that is making artificial hearts. I think the training that students got in my lab was very good in that they get exposed to all these different things we're talking about. It's very broad.
ZIERLER: Right.
BELLAN: They get used to jumping into different areas. One is working at NASA on reentry dynamics. When a spacecraft comes in, it burns up. It has a heat shield that gets real hot and oblates. This has been an issue. They have simulation chambers for that. That's essentially a plasma problem. He's working on that. I think they're in a very broad range of fields. One is working on antimatter and making positronium atoms or looking at positrons and electrons interacting with each other. That's kind of a plasma physics, where the positive particles a positron rather than an ion. That's more basic physics.
ZIERLER: Paul, in terms of the kinds of students who are attracted to your research group, what are some commonalities in terms of their undergraduate focus and their interest generally in science?
BELLAN: I don't think, I'm not sure if there are commonalities. I think they come from a multitude of backgrounds. I think that maybe the commonality is that they're not quite sure what they want to do, and they're looking around to do something different. Because most people don't know much about plasma physics when they come. When they start, it's not something that's widely taught.
ZIERLER: For you, what kinds of interactions have you had with undergraduates over the years, and what courses have you taught?
BELLAN: For many years I taught an undergraduate lab that was a compendium of experiments contributed by faculty. That got shut down a few years ago. I've had undergraduates work in my lab as SURF students for many years. Some are research projects with varying success. Some of them have been terrific, and others didn't do much. But I'd say my undergraduate interactions have been largely from the summer internships. Some of them will continue to work in the lab during the year too.
ZIERLER: Paul, finally, last question for today, just a snapshot in time, circa March, 2022, what are you working on these days, what's most interesting to you?
BELLAN: I'm working on several things at once. I'm often doing that. We have a new ice dusty plasma experiment that is just coming on line. It's been severely delayed because of COVID, my students couldn't work in the lab. But at least it's working a little bit, and we're collaborating with Murthy Gudipati from JPL, who's an astrophysical ice expert. We're setting up an experiment to measure the infrared spectrum of ice in the plasma. It's pretty neat to have something that's really cold and really hot at the same time. We're trying to be cold and hot at the same time. We have cryogenic stuff in plasma at the same time.
Then, we're trying to understand this analogue of blowing bubbles. How does the plasma break off. We've been doing that for years, and we're at a new level of sophistication on that. I have people working on that. Then, in astrophysics we've got some new projects involved on what's the mechanism by which accretion disks provides the power for an astrophysical jet. We have active work on that, so there's quite a bit going on.
ZIERLER: Paul, this has been a great conversation. I'm so happy we were able to do this. Next time, we'll go back, learn about your childhood, educational trajectory, and your career at Caltech.
[End of Recording]
ZIERLER: This is David Zierler, Director of the Caltech Heritage Project. It's Wednesday, March 23rd, 2022. I'm delighted to be back with Professor Paul Bellan. Paul, great to be with you again, thanks for joining me.
BELLAN: A pleasure to be with you, David.
ZIERLER: Paul, to start, today we're going to go all the way back to the beginning. In our first conversation, we did a wonderful tour of your approach to plasma physics and some of the big questions in the field. Let's start first now with your parents. Tell me a little bit about them.
BELLAN: My parents, oh. My father and mother are from Winnipeg, in Canada. They grew up there. I guess my mother grew up partly there and partly in Saskatchewan. My father went off to university there, and he was in the Canadian Air Force during the Second World War. Was in Burma. My mother worked for the British Admiralty in New York during the war and had some interesting stories. She would arrange for accommodations for sailors who were in convoys, who'd come across the Atlantic and were from England, never been to North America, didn't know New York, and she would set them up with places to stay and what to do. That was kind of exciting for her.
ZIERLER: Paul, did your father ever talk about his wartime experiences?
BELLAN: A little bit, not too much. I wish I'd asked him more. He was in Burma and I remember him talking about the Japanese dropping presents on him, that's the way he put it. I think he had a pretty interesting experience during the war, but he didn't talk too much about it. I wish I'd ask more.
ZIERLER: What was his profession after the war?
BELLAN: He became a professor of economics. He was fairly distinguished. He wrote some textbooks and served on some government committees, wrote articles in newspapers and had many students. Occasionally I'll bump into people who say they took classes from him—
ZIERLER: Oh, wow.
BELLAN: —over the years, so that was always very nice.
ZIERLER: Now, did he pursue a graduate degree after the war?
BELLAN: Yes, yes. He got a PhD at Columbia University, in fact. I was, I don't know, 2 or 3 years old, we lived in New York. While he was a graduate student at Columbia, I think I slept in a closet because he didn't have much money for an apartment.
ZIERLER: Do you know, did Canada support veterans education the way that the United States did after World War II?
BELLAN: I think somewhat. I don't know exactly. I know there was a big boom in education after the war with all the veterans coming back and wanted to go to university, so it was kind of a golden age for universities.
ZIERLER: Did your parents meet in New York?
BELLAN: No, they met in Winnipeg. I think they met in Winnipeg on a double date, where they were not dating, they were dating somebody else.
ZIERLER: Yeah.
BELLAN: My mother went back to New York and then they corresponded, and I think because of the letter writing, they got together, got engaged, and got married.
ZIERLER: Did your mom work outside of the home when you were growing up?
BELLAN: Yes, my mother was very advanced in that regard. She was trained as a psychologist. She didn't work for people, she was working by herself, but she became an expert in teaching people with dyslexia over the years, and developed her own methods. She helped many, many people to learn to read. There's a whole string of people who I've met over the years who were grateful to her for teaching them to read. She wrote a book on teaching your child to read that was sold for a while.
ZIERLER: Paul, where did you grow up?
BELLAN: In Winnipeg.
ZIERLER: Is that where your father taught?
BELLAN: Yes, I didn't go away to college. I lived at home and commuted to college. In Canada, in those days and I think still, there really wasn't the tradition of people going away to colleges. Go to university in your own city, mainly. I think it's gotten a little bit more like the US, but not so much. I just lived at home and carpooled to university. That has its advantages and disadvantages. I could see that they kind of balanced out in the end, because you didn't get the growing up experience of going away from home. That got postponed for me until I went to graduate school. But then, you had the advantage of living at home, and this big financial business of American universities, especially private ones, where there's lots of money involved, didn't exist there. The tuition was, wasn't free, but it was almost free. It was next to nothing. The tradition then was for students to work during the summer and summer jobs, which I did. You'd save more money than you needed during the year, so usually by the time you finished your last year, after you graduated, you had a lot of money saved up. The tradition then was to blow it all on a trip to Europe, which is what I did, and many of my friends did that. Whereas my American compatriots were always in debt at that time, and the last thing in their mind was to go and spend three months traveling around Europe.
ZIERLER: That's a much better model, if I might say. Paul, growing up, were you always interested in science?
BELLAN: I think so, yes. I was a kind of a stereotypical nerdy kid who was interested in radios and electronics, reading everything I could about that. I discovered a lot of my colleagues at Caltech were like that too when they were young. I was a ham radio operator.
ZIERLER: Did you go to public school throughout?
BELLAN: Yes, although I had one very interesting educational aspect. My dad took a sabbatical in England at the University of Manchester, when I would have been a senior in high school. I lived in Manchester, England then. My father asked around and found that there was a very good grammar school there and he got me enrolled in that. It turned out, that was one of the top schools in England. I spent a year there, and that was very informative for me. I mean, I was always a very good student, but that, I'd say, gave me a boost in a lot of ways, so that when I came back home, I went to university as a freshman, but I think I was way better prepared than everybody else because of that year in England. I guess I had to go through a lot of challenges adjusting to that year in England, so coming back home was kind of easy. Other people were going to university and they experienced all the difficulty of going to university, and for me it was like coming down from a challenge to something that was easier. That was quite important in my life, that experience. We travelled all over Europe. We spent three months before the academic year travelling around the road for three months, and then the second year we travelled all over Europe. I travelled way more than just about anybody I know, certainly at that time.
ZIERLER: Wow.
BELLAN: All that traveling was pretty influential in my life too.
From Winnipeg to Princeton
ZIERLER: Paul, what year did you enter university in Winnipeg?
BELLAN: I guess it would have been 1965, the fall of '65.
ZIERLER: At that point, were you already set on pursuing an education in physics?
BELLAN: Yes, I entered in the physics program. Then I think you already specialized in physics at that point, I think. I'm pretty sure I had already selected physics. I think I took physics, chemistry, mathematics, French, and philosophy. The French I didn't have to take, but because I'd spent the previous year traveling around in Europe, I figured learning a foreign language was a good deal. Growing up in Canada, I was forced to learn French in school, so I took many, many years of French, and didn't get very good at it. But then, when I came home, I decided, hey, I have this head start in French, better take advantage of it. I took French and improved it and got reasonably decent at it.
ZIERLER: Paul, looking back, what aspects of your undergraduate education emphasized theory, and which emphasized experiment?
BELLAN: There was very little experiment, and in fact I took a lab—actually there were two or three labs. I took an optics lab, and I remember that was the worst grade I got. I forget what I got, but maybe I got a D in that or something. I was surprised at that. Then I think there was an electronics lab, which I did very well in. I discovered some kind of ringing that the professor didn't understand and figured it out, and he was very impressed that I figured that out. But I was also doing a lot of electronics on my own. I was interested in high-fi and stereos, so I built amplifiers and speaker systems and circuits. Then I was a ham radio operator. I built a transmitter and strung up antennas, so I was pretty much involved. I went to science fairs.
Something I was kind of proud of was, I built a television camera when I was about 15. I got the tube for that from a TV studio I visited and spoke to the engineer, and he gave me some worn out vidicon tubes. Then I found a magazine, Popular Electronics or something like that, that explained how you could make the circuit for that. There were circuits needed to scan the electron beam in that, and I really wasn't up to making that at 15. I had the idea that I could get a regular TV receiver, the kind you watch, and it also has circuits for scanning, so I stole the signals from that, and ran my vidicon with that. I got it to work just marginally. Something was wrong with the amplitude, so it didn't work well. However, I was able to take a wire and move it in front of the vidicon and you could see on a screen something move back and forth in a blurred way, so I considered that I had succeeded in getting video. But that was maybe one step above not working at all, but it did provide an image that moved. That was part of my playing around with electronics.
ZIERLER: Paul, as an undergraduate, did you get exposed to plasma physics at all?
BELLAN: No. No exposure at all. What happened was, I read an article in Scientific American that—this was talking about tokamaks. I haven't found that article, but I remember it showing the plasma being held in a magnetic field and being intrigued by the idea that you could have something suspended in a magnetic field. That seemed interesting. Then, I guess I did some research into what I would do afterwards, and at University of Manitoba, people were doing either nuclear physics or solid-state physics. They had a small cyclotron there and then a lot of people doing solid-state physics. I remember sitting in a library and looking at the journals in those subjects and there were shelves and shelves and shelves of that. I felt that if I went into those fields, I'd just make the shelves a little thicker. That there was already too much. I wanted to go into a field that wasn't so developed. I also was interested in the energy aspect of plasma physics and fusion. Because of that research, I decided to get into plasmas, but I didn't know anything about plasmas.
ZIERLER: What does that say about the field or your curriculum, that plasma physics was not on your radar as an undergraduate, at least officially?
BELLAN: No, nobody mentioned it to me. I sort of found out about it myself.
ZIERLER: No, but the question is, is your sense that plasma physics during those years was a backwater?
BELLAN: I don't think it was a backwater, it was just a very small field compared to other fields. The number of people working on it was tiny compared to others, so nobody knew about it. Maybe at some other universities, there was something. In Canada, I mean in retrospect, I know there was some things going on out at the University of Toronto and the University of British Columbia. There were people interested in plasmas regarding spacecraft, because the Canadians at that point had started building spacecraft, and putting probes in spacecraft to measure plasma waves. I didn't know about any of that then. There also was a rocket range at Churchill in Hudson's Bay, where they were shooting sounding rockets up. There was a factory in Winnipeg actually, that was a premier place for making these rockets. They're called BlackBrants. Those would have been studying plasmas in the ionosphere, I would think, but I didn't know about any of that at the time.
ZIERLER: Paul, toward the end of your undergraduate career, the late 1960s, had the antiwar movement come to Winnipeg? Were there American students there, were there protests on campus at all?
BELLAN: A tiny bit. We weren't insulated from it, we were totally aware of what was going on. There were one or two Americans who had fled the US to flee the draft, who were there, that I knew of. Then there were students who were very much against the war in Vietnam, and then there were some people who were very pro-American. The one incident that I remember that was, I thought, was, in Canada, there wasn't the same level of anti-communism as there was in the US at that time. It was legal to be communist. In the US, you'd get tossed in jail if you were communist or something. Not that there were a lot, but there were some, and you could do it, and there were some students who were that, and there was a handful of politicians like that.
At my university, there was a guy, I still remember his name, I think his name was Joe Flexer or Flexter or something like that, who was the sort of the communist who made a big fuss about it. Then there was another guy who was kind of the opposite, he came from a blue-crust wealthy family that lived in sort of the equivalent of San Marino. The best part of town. He had a name like that, his name was Darcy Bancroft, sounded like somebody out of a novel. He was very much in favor of the fighting against the communists in Vietnam. Somebody decided to organize a debate between these two guys, and of course everybody went to hear them. Bancroft was sort of spouting the American line, and Flexer was spouting the anti-American line. It was all kind of stereotypical stuff. But one of the bones of contention then, was the US was talking about the Ho Chi Minh Trail and saying that the Vietcong were a fiction, they were really North Vietnamese soldiers, who were infiltrating from North Vietnam into South Vietnam on the Ho Chi Minh Trail, and they were like ten thousand a day coming in and that's who they were fighting. He said that every day there's ten thousand North Vietnamese soldiers crossing into South Vietnam, and Joe Flexer said, "OK, name one." That stuck with me.
ZIERLER: Paul, was there a professor or a course that convinced you to pursue physics for graduate school?
BELLAN: I was already deeply into physics by that time. But yeah, the system there was different from here. I think I had enrolled in physics as a freshman, and then after that, they had two streams. There was a three-year degree or a four-year degree, with the sort of standard one being a three-year degree at that time. The four-year degree was called honor's physics. The four-year stream, only a tiny fraction of people took that. Maybe a third or a quarter, something like that. The four-year—all the Canadian universities were like that, I think. The honor's physics program was really at the level of the program that you'd have at Caltech or Princeton or MIT or something like that. I think that started in the second year, and I took honor's physics, so I had a very good undergraduate education in physics. The first year I took French and philosophy, and after that I took no humanities classes. It was all mathematics and physics for the next three years. Caltech students actually get a broader education than I did, because they have to take a humanities class all the time. Every term they're supposed to take one, but I didn't do that.
ZIERLER: Paul, did you get advice that you should focus on graduate schools in the United States specifically?
BELLAN: Not from anybody at the university, but I had a relative who was married to a cousin of mine, who was very familiar with the American system. He had obtained a PhD in physics at Brown University, and he went on to become a physics professor at the University of Victoria. He encouraged me to apply to the usual gang of American universities, which I did.
ZIERLER: Why Princeton in the end?
BELLAN: They were the premier place for plasma physics. That was the obvious place to go if you wanted to do plasma physics.
ZIERLER: This was really you pursuing your interest in plasma physics independently, that convinced you that this was what you wanted to focus on for graduate school?
BELLAN: Yes. Yeah, nobody, I don't think anybody advised me to do Princeton. I researched it and decided that was the place to go.
ZIERLER: Who was at Princeton at that point that attracted you in plasma physics?
BELLAN: I didn't know anybody there. I just knew Princeton was the place for plasma physics.
ZIERLER: Just by reputation.
BELLAN: Yeah, this business of students visiting ten universities and looking at websites and reading US News and World Reports, none of that existed. We were totally naïve about all that. I had no idea what I was jumping into. I didn't know of the people, I didn't know what they were doing, I didn't know what the subject consisted of, or anything. I just dived in, and that was that.
ZIERLER: Paul, I asked you about the social scene in the late 1960s as an undergraduate. What about when you arrived in Princeton in the early 1970s, was the antiwar movement strong at that point too?
BELLAN: Not too much, I don't think so. Certainly not at Princeton. There was a tiny bit, but I wasn't aware of much. I don't know. I remember some of the students being in graduate school because they didn't want to be drafted. I remember that there was quite a difference in politics at the lab, depending on the kind of profession people had. I was at the Princeton Plasma Physics Lab, which was kind of like JPL is connected to Caltech. It was off-campus thing. It was a little bit more academic than JPL is, in that there were professors there and you could be a student there, but it was essentially a national lab type of thing, where, probably at that time, there were maybe 500 people working, of which maybe 10 or so were graduate students and the others were professional scientists or engineers or technicians. The PhD scientists, I think, were all antiwar, or at least most of them were. The engineers were maybe mixed, and the technicians were all probably pro-war. There were these three types of—if you think of the kind of polarization we have now, there was that kind of polarization there, but you could see it at the lab.
ZIERLER: Certainly, certainly. Paul, how well prepared were you, looking back, relative to students that might have gone to bigger schools for graduate school?
BELLAN: I was extremely well-prepared academically. They had a first-year exam, it was called the prelim, that you had to take, and I think I got the best grade in it. Then I decided not to take the first year quantum class and instead jump into the second year one, which I did okay in, and then I regretted, that I thought I should have taken the first year one, to review quantum and get it down. Actually decided to take the exam for the first year quantum class, even though I hadn't taken the class, just to see how I'd do, and I did fine in it. But I had, I think, excellent preparation. Although I didn't really feel that at the time so much, because there was another kid from Canada who'd gone to McGill University, and he had taken an engineering physics curriculum that was much heavier than I had. He was much better prepared than I was, so I was always sort of looking up to what he was doing. But I think we were both doing very well.
ZIERLER: Paul, surveying the department in its entirety, in the early 1970s, what were some of the most exciting happening at Princeton in physics at that point?
BELLAN: I didn't know what was going on in physics much, I just knew what was going on in plasma physics. There was a bit of a bubble there, because, I mean, I barely understood what was going on in plasma physics. I was struggling to learn this field and to keep up with it. It was an exciting time in plasma physics certainly. You're probably not familiar with that, so I'll tell you the story.
ZIERLER: Please.
BELLAN: I guess, you go back to Hiroshima, there was the atom bomb, and then after that, there was the hydrogen bomb. People had made fission reactors that used the same principle as the atom bomb, and then the question was, whether you could make a fusion reactor, that would be like a hydrogen bomb. The mechanism is very different. Efforts started in the US and Russia and the UK in the late 40s to do that, and it was all top secret, because it was assumed to be connected to bomb research. People worked on it and didn't really get very far, and by 1958, they realized that they weren't going to get very far and furthermore, it had no relation to military anything. The three countries got together and decided to put all their cards on the table and have a peaceful cooperation. There was a conference in Geneva in 1958 where everybody gave talks on what they had done.
The US had a device called the stellarator, which had been invented by Lyman Spitzer. Caltech has a Spitzer telescope, that's the same guy. He was an astrophysicist, who started the Princeton Plasma Physics Lab. The stellarator was a very elegant thing, but it didn't work very well. They pursued it up until the 60s and with some progress, but they were getting nowhere near what you needed. Then the Russians had something they called the tokamak, and they claimed in the, I guess, middle 60s or so, around '65, '67, something like that, that they had much better results than the stellarator. Nobody believed them, because they didn't believe the Russians, and they thought the Russian diagnostics were too crude. The Russians invited a British team to use a laser diagnostic to measure the temperature of the device, and the British said, yeah, the Russians are right, it's working twice as good as the stellarator or so. The Princeton people tore apart their stellarator and turned it into a tokamak, which took about a year. That was about a year before I came. Then they started building bigger and bigger tokamaks, and the whole field kind of exponentiated. They're still building bigger tokamaks. There's this one ITER in France, I'm not sure if you've heard about it.
ZIERLER: Sure, yeah.
BELLAN: It's kind of just a continuation of that. That's on the verge—that should have fusion in a few years. I came there around the time that this whole thing took off, and I was at least somewhat aware of that. I was always doing fundamental stuff, so I wasn't working on these parameter pushing machines, but I was next door to them.
ZIERLER: Once you got the lay of the land in the department, who was working on plasma physics among the faculty?
BELLAN: I was in a place where everybody was doing plasma physics.
ZIERLER: Uh-huh.
BELLAN: The plasma physics lab was essentially a department of plasma physicists. It was organizationally an offshoot of their astrophysics department, so the astrophysics department was in a little building on the Princeton campus, with, I don't know, probably eight professors in it. Then, on an organizational chart below it said plasma physics lab, and this plasma physics lab had 500 engineers and technicians, and we'd be another dozen or 20 physicists on it. It was a critical mass. The courses I took were all in plasma physics and I was just taught by people doing plasma, who were plasma physicists.
ZIERLER: Of course, this is before the DoE. Was the AEC, the Atomic Energy Commission, were they supporting this?
BELLAN: Yeah, this was the AEC at that time.
ZIERLER: Was it an FFRDC, was it basically a federally supported lab?
BELLAN: Effectively it was. It was sort of like JPL is to Caltech, but it was part of Princeton University, but paid for by the federal government. It had more government-type aspect to it. There was some more security there than you would find in the university, and more bureaucracy. The graduate experience I had, I think, was kind of bizarre. It took me a while to realize that it was bizarre, because at a normal university, the professors have graduate students, and the graduate students are the workers for the professors. Like Caltech runs on the work of the graduate students and the postdocs. At the Princeton Plasma Physics Lab, they didn't need graduate students. They were just sort of an inconvenience they put up with, and so we could have all disappeared and nobody would have noticed. They weren't depending on us in the slightest. It was just, we were being supported out of altruism. They figured there should be a next generation of people, they should educate somebody, but nobody was depending on our work.
ZIERLER: Was there a national security component to the lab at all, was there any classified work going on?
BELLAN: No, no. There had been early on, because in the library, I'd look up things in the library, and there were reports from the 1950s that all had stamped ‘classified' on them, and then that was crossed out, that said ‘declassified', with somebody's signature on it, on every page of the report. But when I was there, nothing was classified. It was all open.
ZIERLER: What were the big projects, what were you able to slot in on from the beginning?
BELLAN: Again, I wasn't really on big projects. The big projects were these tokamaks, which were machines that would have a crew of 10 or 20 PhD physicists working on them, and a similar number of engineers would take five years to build and would cost tens of millions of dollars, and eventually a billion dollars. There were some graduate students who worked on those, but you'd just be a minor cog in a big operation. I guess, the closest thing I can think of, would be to be like a graduate student on the James Webb Space Telescope or something.
ZIERLER: Wow, that's big.
BELLAN: You'd get some time on it, but you wouldn't really control how the thing's being used. I worked on small machines where I, in some cases, more or less built the machine myself and I didn't have any competition, I had complete control over it. It wasn't so different from the kinds of devices we have at Caltech. These are machines that would, I don't know, I guess nowadays cost a few hundred thousand dollars to build something like that. But it wasn't a multimillion-dollar thing.
ZIERLER: What were some of the theories or the bigger research questions that were driving the experiments in the lab at that point?
BELLAN: I think there was a big disconnect between experiments and theory in plasma physics at that time. You can generate lots of theory in plasma physics, there are beautiful equations and there were theoretically inclined people who would do all sorts of models, and you could spend your life on that. But then when people did experiments, they observed things that made almost no contact whatsoever with these theories. I guess, if you're not a scientist and you think of what scientists do, you think, oh, they make precise measurements, they measure something that's 503.142, and you get the next decimal point or something like that. Or holding a test tube up and looking at it. Plasma physics, a lot of it, nobody had a clue what was going on, and you didn't know whether the density was a certain value or ten times its value or a hundred times its value. If you could get an answer that was within a factor of 2, then you considered you'd made progress. There were all sorts of theories of plasma waves, different kinds of waves. In those days, people were just trying to observe that these waves existed, that you would see something that was consistent with the theory. That's the kind of thing I did when I was a student, try to generate a certain kind of wave, because the generation mechanism was very non-intuitive. It wasn't at all obvious how to do it, so you'd make an appropriate structure for generating it, and you had to measure it, and then if you could make connection with theory, then that was a big success. That's the kind of thing I did, and other students of that vintage were doing too.
ZIERLER: Paul, when you say that this was an exciting time in plasma physics, it was exciting because it was foundational. There was so much to discover.
BELLAN: I think so, yes.
ZIERLER: What was the process of developing what would become your thesis research?
BELLAN: It happened in a very disorganized way. In fact, the way it happened has kind of influenced my thinking since then, and I told you last time about being opportunistic, and this related a little bit to that. Again, I was very naïve about everything then. I didn't understand the politics or how research worked or anything. I was just passing my exams and studying and when I got to the point where I had to do research, I was assigned to work with a group that was looking for a certain phenomenon. As I said, the students were somewhat unneeded there, so I was assigned to this group, and I don't think they were terribly happy that I was with them. They were trying to see this wave, and they were putting an antenna on, and they saw some little blip and claimed that they saw it. I wasn't getting along very well with these people. I mean, there wasn't anything nasty, but they just weren't interested in me participating very much, and I didn't know how to work my way in. I wasn't so happy, and they weren't so happy.
Then, I guess I don't really know how this happened, but the people above probably realized that this wasn't working out, and they suggested that I transfer to have another advisor, who was, I think, much more theoretical than these guys were. He took me on. Then I started thinking about what they were doing and also—I guess when I switched, there was concern about what I would do. As example, what would be my thesis project. There was concern that I would duplicate what they were doing or compete with them, because I had learned what they were doing and I go work for this other person. I was supposed to write down what my plans were, to make sure that they didn't overlap with them. It was like a non-compete clause. I remember, I spent a day and I wrote down my plans for my thesis, that I do this, that, and the other thing. I guess I showed it to people. Anyways, I filed it away, and then when I graduated, in cleaning out my desk, I found this plan, which I had forgotten about. When I saw it, I started laughing, because what I did was completely unrelated to that. It was complete garbage. That's when I concluded that planning is a bit of a waste, and this thing, that you should just get involved and see what happens. I was inspired by what this group was trying to do. I started thinking, doing some theory, and I'm not sure quite what started me on it, but I concluded that they were doing it the wrong way. There was a phenomenon called a resonance cone. You're familiar with Dirac delta functions?
ZIERLER: Yes.
BELLAN: A Dirac delta function can be written as an integral over cosines. So, everybody's working with dispersion relations, and so they're assuming everything goes like cosine kx – omega t, and they assumed that if we put an antenna in, it would excite something that goes like cosine kx – omega t. But, for this particular wave, if you put an antenna in, it's like a point source in space, so it's like a delta function. It doesn't excite a single k, it excites a whole spectrum of k's, and they superimpose to give you something like a delta function, because it's a point source. Then what happens with these waves, is that the delta function itself kind of propagates along in space, so instead of having ripples spreading out, which is what you'd think a wave is, you have this delta function, this thing that's trying to be infinite. In the simplest approximation, it's propagating away at an angle relative to the magnetic field, because you're summing up all these cosines that are cancelling everywhere except that one place where they all add up, and you get this infinity.
I figured that out, and then I discovered other people thought of that too. There was a group at MIT had done that, and Roy Gould at Caltech had done that, with a student. But I sort of independently realized that, and I realized that if you wanted to see a cosine dependence, which was what the theory predicted, then you better have an antenna that has a cosine dependence also. You'd have a periodic antenna that is a phased array that puts on a well-defined wave vector, and then you can see that. That was my thesis, and I was able to show this wave satisfied the dispersion relation because you were forcing it to have a cosine dependence. If you just put in a single probe, which is what people were trying before, you didn't get that.
ZIERLER: Paul, would you say, as we talked in our previous conversation, the way that you've mixed experimentation and theory, does that go all the way back to graduate school days, in terms of your approach to plasma physics?
BELLAN: I think so, yes.
ZIERLER: What were some of the key points or conclusions in your thesis that might have been responsive to larger questions in the field at that point?
BELLAN: I don't think I would say I was thinking that big. I was just working at my own thing. But this stuff I did on these waves was moderately influential. My thesis advisor, Miklos Porkolab, went on to MIT and became a director of the plasma lab, plasma effort at MIT. He always pushed these ideas and people continued working on that, and were seeing this phenomenon that I had seen in a much more detailed way. I think that had some influence on at least a certain subset of people working in fusion.
ZIERLER: Paul, thinking about to the extent you were in a plasma physics bubble, as a graduate student, were all of the fantastic advances in particle physics, did that register with you at all as a graduate student during the early 70s?
BELLAN: No.
ZIERLER: It really was a bubble.
BELLAN: There was really a bubble, yeah. I was struggling to understand what was going on in plasma physics.
ZIERLER: Yeah.
BELLAN: It was a whole world that I didn't know. I mean, even then, there were huge parts of plasma physics that I wasn't aware of as a graduate student. All the things having to do with space physics and astrophysics and solar physics I had no idea about at the time.
ZIERLER: A real bubble indeed.
BELLAN: Yeah.
ZIERLER: Paul, who was on your thesis committee?
BELLAN: Oh, wow. I'm not sure. Obviously, my advisor and Martin Kruskal, who was one of the stars of the applied mathematics and the plasma physics, who was perhaps the smartest person I've ever met.
ZIERLER: Wow.
BELLAN: I'm not saying he's the smartest person in the world, but he's the smartest person I ever dealt with. I probably had Tom Stix on it and maybe Carl Oberman. I can't remember who it was, but these were the people who would have been on most committees at that time, so they probably were there.
ZIERLER: Anything memorable from the defense?
BELLAN: I think I was asked a question that, of course, I couldn't answer, that I'm not sure I'd even know the answer now, by Martin Kruskal. I have to tell you a little bit of plasma physics for that.
ZIERLER: Please.
BELLAN: In a certain limit, plasmas are collision-less, but you get behavior that sort of emulates collisions. It means that entropy is conserved. There's no friction. The system is completely deterministic and yet, it can behave in ways that look as if there's friction, even though there isn't friction. What's happening is that, you get what appears to be randomization, but it's just confusion develops at the microscopic level, and if you're clever, you can unravel the confusion. The analogy I have, I've thought a lot about these things, so I've come up with analogies, so when it's supposed that little green men landed in flying saucers on earth in 1600 and left some holograms, and that you could only view those holograms with a laser, and so people would look at that and they wouldn't see anything. When people studied entropy, they'd look at this and they'd say, it's completely random, this is a high-entropy thing. There's no information in it. But then, the 20th Century comes along and lasers are invented, and they shine a laser on it, and they find that this is an encyclopedia of knowledge. There's a huge amount of information in it. The information is there, but it's all garbled in the microscopic sense. If you've got the correct key, like the laser, you can unravel it.
Then the question, the philosophical question is, if you're in the 1600s, were you entitled to say this was a high-entropy thing that has no information on it, because lasers hadn't yet been invented, and so you didn't know any better, and so as far as you are concerned, there's no information there. But then later, there was information. I came up this idea that entropy is a subjective concept, it's in the eye of the beholder. If you have the laser, there's no entropy. If you don't have the laser, then there is entropy. Plasmas kind of do that spontaneously. You can have plasma waves that appear to decay, and they're effectively turned into holograms that you can't see. But if you're clever, you can do the equivalent of shine the laser on it, and then you can get it back again. In my exam, I was asked about a particle that'd be moving along and making a wake of waves, just like a boat leaves a wake, and then these waves would disappear. It looked as though they died away. Because this is collision-less, and there's no entropy production, you can run all the equations backwards in time. I was asked, what would things look like if I ran the equations backwards, because it seems like it's a one-way thing, that entropy is increasing, but really it isn't, so how do you have a wake—wake look when you run time backwards; I'm not quite sure what the answer to that is still, but that was the question.
ZIERLER: Paul, after you defended, what opportunities did you have, what did you want to do next?
BELLAN: I had, again, a kind of anomalous situation, where my advisor went off for a sabbatical in Germany for a year. So, I don't know how this was decided, but it was decided that I would be a postdoc and stay on in the lab, with me being the boss, because there was no advisor left. I kind of took over the lab, as a postdoc, and ran the show for a year, and continued doing similar things to what I was doing as a graduate student. I became a member of the executive committee of the lab, even though I was a postdoc, because I was representing this group. That's what I did, and then after that I came to Caltech. So, I had a somewhat unusual postdoc.
ZIERLER: What was your research focus during your postdoc year?
BELLAN: That was just extending the kind of stuff I did for my PhD. I was studying some different kinds of waves. I was still interested in resonance cones, and I discovered that the resonance cones I had studied were high-frequency ones, that were frequencies sort of above the ion plasma frequency, and above the ion cyclotron frequency. I proposed that there be a rather different kind of resonance cone at much lower frequencies, and I made an experiment and I saw it. Kind of unhappy that people haven't paid more attention to that. Some people have, but I really saw this thing and it worked.
ZIERLER: What was the significance of that?
BELLAN: Basically, dispersion relations can be no good in reality. That if you put a localized source in, it excites a whole spectrum of waves, and they add up in strange ways, so that you get things that are very non-intuitive. This is related to the thing I mentioned last time, where the group and phase velocities are orthogonal to each other. Any intuition you have about from dispersion relations is essentially wrong, because everything goes perpendicular to what you think of that way. Basically, when you do dispersion relations, you're doing the Fourier transform of the equations, and to find out what's going out in the real world, you've got to the inverse Fourier transform. I felt that physicists got lulled into thinking of Fourier space too much. When you do quantum mechanics, you talk about hbar k all the time and wave vectors, but the real world isn't wave vectors. That's a Fourier mode, and you have to add up a bunch of Fourier modes to get back to the real-world situation. I think people were never doing that. They were always working in Fourier space and forgetting that we don't live in Fourier space, we live in real space. Real space could actually look very different from Fourier space.
ZIERLER: Paul, did you have the offer from Caltech before the postdoc, or you were on the market for that year?
BELLAN: I never really went on the market. I don't remember the details, but I don't think I was looking too hard for a job. I think I was looking a little, but I think I got the offer without really looking for it too much.
Roy Gould and Arriving at Caltech
ZIERLER: What was the circumstance of the recruitment? Was Caltech looking to build its plasma physics component?
BELLAN: Yeah, I think Roy Gould wanted to hire a young plasma physicist, and I think he interviewed a few people. I don't quite remember how I got into it, but I think I heard he was looking for me, and maybe I applied then. It's not like nowadays where people advertise and you apply for hundreds of jobs and you fill out all your statements saying how you're following all the things you're supposed to follow. I didn't know about any of that stuff.
ZIERLER: Paul, in the way that you were attracted to Princeton because you appreciated that it was a center for plasma physics. Was it the opposite at Caltech, that your hire represented an effort to build up the program?
BELLAN: I think so. I'm not sure. I think Roy Gould wanted to hire another plasma physicist and got permission to look for somebody. Again, things weren't done the way they are now. It was much more informal. I don't know what went on behind the scenes frankly.
ZIERLER: What was Roy Gould working on at that point, when you joined Caltech?
BELLAN: I think he had just built a small tokamak. As I said, tokamaks came on the scene in the late 60s, and they were big machines that a place like Princeton would have a sort of national lab type thing where you'd have 20 people working on it. In today's money, the starting point would be maybe 10 or 20 million dollars for the beginning. Then, there was a guy at UCLA, Bob Taylor, who had a real green thumb experimentally. I think he had been at MIT for a while, and he advised them in building a fairly significant big tokamak, and then he came to UCLA. He came up with the idea of making cheap, university-scale tokamaks that didn't cost very much. They didn't compete with the big ones, but they still worked as tokamaks, and you could do a lot of interesting basic physics on that. He had a bit of a cottage industry, where he was building these and either giving them or selling them to people. I think Roy, I think he built his own, but under the tutelage of Bob Taylor. They were called Taylor tokamaks. He had just built that around the time I was there. Then I came and I built a tokamak too. But the tokamak I built was quite different. I didn't want to copy what other people were doing, so I had my own tokamak that was kind of unique.
ZIERLER: How did you build it in a unique way, what was different about it?
BELLAN: The standard tokamak was a pulse-machine, that would make a pulse once every two minutes or five minutes, and which would have fairly intense parameters. The goal was to make a hot plasma. There's some kind of threshold, you'd have to put enough power to go over this threshold to get a proper tokamak that would be hot enough and interesting enough. But then it was hard to diagnose, because you only had a shot every few minutes, and it was very hot, it would burn things up. I had the idea of making it a machine that would not be very ambitious in terms of parameters. It wouldn't be very hot. It wouldn't have very high currents. It would be totally wimpy. But it would have a very high repetition rate. Instead of a one shot every five minutes, it would be operating at fifteen shots per second. It was almost like a steady state device. Then because of the modest parameters, you could stick probes in and they wouldn't get burned up, and so you could make all sorts of fundamental measurements on it that wouldn't be possible on other tokamaks. Because you had the time, you had lots of shots, it was almost like a steady state thing. You could move a probe slowly through it and measure something as a function of position, which wouldn't be possible in something which just had a shot, one shot every five minutes, and it wouldn't burn up the probes. There's a whole class of interesting things you could do and that's what I did.
ZIERLER: Did you take on graduate students right away?
BELLAN: Yes, I think so, yes.
ZIERLER: Was the goal to build a larger group or plasma physics was always supposed to be a small endeavor at Caltech?
BELLAN: I'm not sure what it was supposed to be, but I don't think there was a goal to build a smaller group. I think it was just to have something going on.
ZIERLER: What were some of the funding sources at Caltech in the early years?
BELLAN: I had to write my own proposals. The money wasn't coming through Caltech at all. I was getting money alternately from the Department of Energy or the National Science Foundation for many years. Sometimes they had a joint program. I'm still getting money from them, but in recent years, it's expanded to some other organizations too.
ZIERLER: Did you collaborate with Gould?
BELLAN: Not directly. We never worked on a project together. I think he intimated to me that he didn't want me to collaborate in a sense. He wanted me to be an independent person, as a beginning professor. He said it was important for me to have my own independent research activities and so if I collaborated with him, then I would be considered to be his sidekick. He didn't want that. I tried to do things that were independent. I did things that were independent. But we had joint seminars. His students would talk and my students would talk. He would serve in my students' exams and I would serve on his students' exams. We might borrow some equipment from each other. I'd ask him for advice for things. We discussed things a lot, but we weren't working on the same project at any time. We didn't share funding.
ZIERLER: Your work on tokamaks at this point, were there ambitions for applications? Were you thinking about nuclear fusion?
BELLAN: Tokamaks, yeah, this is related to nuclear fusion, but I was trying to do fundamental things that would be relevant to tokamaks. My little tokamak had tremendous accessibility for things. A big tokamak, if you're a student, you'd spend a year or two building a diagnostic and then they'd give you a week to use it on a machine, and you collect data for that and then you're supposed to go back and analyze it. In my little tokamak, although it had nowhere near the parameters of a big tokamak, it was like a model airplane compared to a real airplane, it did have several aspects that were relevant to tokamaks. It had the helical magnetic fields. It had the magnetic confinement, same mechanism of confinement as a big tokamak. Some of the same instabilities. There's a lot you can study on that, that was relevant. Several of the students who worked on my little tokamak went off to work on big tokamaks at the National Labs, and they had a very good training for that.
ZIERLER: Speaking of the National Labs, I'm curious, did the creation of the Department of Energy change anything for you, in terms of your research funding or agenda?
BELLAN: I don't think so. I think that all these changes of names were totally cosmetic.
ZIERLER: I wonder if you can explain why you publish so frequently in the journal Physics of Fluids, why Physics of Fluids?
BELLAN: That's historical, because that was the journal where plasma physicists published initially. It was fluids and plasmas. At a certain time, I don't know, 30 years ago or so, they decided to split the physics of fluids in two, where one part would be fluids, and the other part would be plasmas. But Physics of Plasmas really is the descendant of Physics of Fluids.
ZIERLER: What was some of your work moving into the 1980s on mirrors?
BELLAN: On mirrors? I don't think I really worked on mirrors except—
ZIERLER: Like a traveling mirror, for example.
BELLAN: On a traveling mirror? Actually, it's interesting, because that's come alive again right now. I'm actually working on that right now. That was a wild idea I had shortly after I came to Caltech. I published a paper that you probably found on it, and I made a little model illustrating it. I tried to convince people to use it, because I wasn't really having a device of the sort that could use it at that time. It came from my work on waves. I had a lot of ideas from waves. You probably heard, or maybe have heard, of the WKB principle in physics.
ZIERLER: Sure.
BELLAN: If you have a wave travel in a non-uniform medium, its amplitude goes up and its wavelength gets short. The mirror confinement consists of two coils with a strong magnetic field near the coils and then a weaker field in-between. It's sort of like a valley of magnetic field and the plasma is stuck in the valley between. I had this idea that you could make the coils part of a wave system, so the magnetic field isn't produced by stationary coils, but the magnetic field is part of a wave that's traveling along something. You have this moving magnetic field. Then you make the velocity of this thing change, so it scrunches up and the amplitude goes up and it gets smaller. That's just what you want for fusion, because if you compress something then you heat it and the density goes up, and if you look at the requirements for fusion, what you want is a high-density and a high temperature. The beauty of this is that it would be spontaneous and didn't require any external energy. It would just be the wave kind of going into a funnel, effectively, and getting scrunched up, because there's a wave moving along. I made a little model to do that.
This idea has been kicking around for many years and people are doing somewhat similar things where they don't have a wave, but they actually just power a bunch of coils in sequence with separate power supplies to make something that looks like a wave. A former postdoc of mine, Sett You, liked that idea, and he set up a company, I guess a couple years ago, where he is going to use that idea for a fusion-based space thruster. I'm involved in that right now. In fact, this morning we just had a discussion on it. Another former student of mine, who's a professor at the University of Maryland, Baltimore campus, is working on it, and they're building the actual coil system. Sett has a lab; he's just rented lab space in Pasadena. He's just setting it up, he's got venture capitalists investing in this. I'm sort of advising, and my postdoc is involved a little bit, and they're building one of these things.
ZIERLER: What are the goals of the program?
BELLAN: You ask me, do I have any ambitious goals, and I said, not really, just following my nose. Sett is different. If this thing works, it'll be a rocket engine that will take you to Mars in a month. It's kind of speculative to say the least, but it's fun.
ZIERLER: I assume JPL is taking an interest in this.
BELLAN: No, it's not involved with JPL, per se. He's got his own stuff. He'll probably talk to people from JPL at some point, he's not against that.
ZIERLER: SpaceX, I would imagine, might be interested.
BELLAN: Perhaps, I'm not sure what he's done with that. But anyways, we basically have duplicated the little coil system that I made 40 years ago, but in a much bigger way. There's serious money into going into making it even bigger yet.
ZIERLER: Wow, exciting.
BELLAN: Yeah, if you want you can look up on the web, his website is helicityspace, all one word, LLC, and you'll see things, and you'll see that I'm listed as an advisor, a scientific advisor.
ZIERLER: I'll take a look.
BELLAN: That's what happened to that idea.
ZIERLER: Back to the 1980s, the mid-1980s, a technical question, what is AC helicity injection?
BELLAN: That's something that I don't really believe in. That was a hot topic, I wrote a paper saying I don't believe in it. I sort of proved why it shouldn't work. If you think of tokamaks, they have helical magnetic fields. I think we discussed this before. You have a straight magnetic field, and then you make a current go along it and that makes a new magnetic field that's wrapped around the original field, and when you add them together you get a helical field. Helicity is a measure of that, and I guess we discussed spheromaks, where you have linkages. That's the same thing. You can think of the straight field with field lines wrapped around that, that would be helicity. Tokamaks have helicity, spheromaks have helicity, and that's the sort of good thing. I guess we discussed, with spheromaks the helicity is conserved better than energy and that's how you get spheromaks. The question is, how do you get the helicity into a system. In a tokamak, you just have a transformer making a current going around that that's effectively injecting helicity.
People had this idea, I came across it in the 1980s, where you could have AC helicity injection, where you'd have sort of a pump. Where you oscillate the field in one direction with the cosine and oscillate from another direction with the sine, and between the two of them, the equations would say that would give you a flux of helicity, and then you'd get a steady state source of that. That looked kind of attractive. I actually did an experiment trying to see it, and I couldn't see anything. I set up the quadrature phase things and nothing happened. I made super-sensitive measurements with lock-in amplifiers, just to see if there was the tiniest bit of a—still couldn't see anything. Then I did some theory on it, and I convinced myself that the way people were using the theory was wrong. I guess this has been kind of a recurrent theme for me, from a philosophical point of view, is that I feel that people often get into trouble where they take a theory and then they use it out of context. They use it where they've not satisfied the assumptions that went into that theory, and they get some really attractive results, but it's no good, because it's been used out of context. I think this happens over and over again.
Anyhow, the problem there with the AC helicity injection, is that you were pumping plasma in and out, and the problem was that there's a wall around. What happened when the plasma hit the wall – this was conveniently ignored. In reality, what happens is when the plasma hits the wall it dies, and then you are kind of making new plasma when it goes in, so it's not the same plasma. It's different plasma. If you're talking about confining a plasma, you're using the word the, which means it's the same plasma. It's not that you're killing the plasma and then making a new plasma and then killing the plasma and making a new plasma, which was what was going on with this AC helicity injection. I did some mathematics showing that this was the problem, and that if you looked at it properly, there wasn't any helicity injection. There's a group at Wisconsin that was pursuing this for years afterwards, and I kept on telling them that it'll never work, it's never work. And, "no, no," they kept on doing it. I think they were claiming to see something, but they never—if this really worked, you would have seen a very clear result that everybody would jump to and say, "oh, we can make money from this," and there'd be big investments in it. But it never had a result that was like that.
ZIERLER: Paul, to clarify, in the non-observation of AC helicity injection, is that to say that you were seeing something else that was being mistaken for this, or you were simply just not seeing anything?
BELLAN: Not seeing anything.
ZIERLER: What are the theoretical ramifications of that?
BELLAN: That AC helicity injection doesn't work. I think I wrote a Physical Review Letter on that, showing mathematically why—what got me going on that is that there's an equation for helicity conservation that was the basis for this. Then, there was a book by a guy named Keith Moffatt in the UK on the earth's magnetic fields, because magnetohydrodynamics also describes the earth's magnetic field and the question is, what's the dynamo that makes the earth's magnetic field. The study of that also involves helicity. It's the same thing. Moffatt wrote this book that is all about helicity and the earth's magnetic field. It was a little hard to follow, because he used a different jargon, and his way of looking at things was a little bit orthogonal to what I was used to. But in his book, he had a helicity conservation equation that was significantly different from the ones that the plasma physicists were using. I couldn't understand what the difference was, and then I finally figured it out. It was essentially a Eulerian Lagrangian difference. The plasma physicists assumed you had a fixed box with plasma in it, and he was assuming that you had a fixed amount of plasma that was moving around in a vacuum. When you talk about the plasma, it's different. I mean, you could talk about that, say, like the population of the United States is the people, but 100 years later, if you say the people, it's not the same people, it's different people. What you mean by the people, if you start talking about the wealth of the country is conserved over 200 years, different people have the wealth, it's not as if you get to stay rich for 200 years. It doesn't work that way. This kind of a confusion was going on.
ZIERLER: Paul, a nomenclature question, we've talked about tokamaks, we also have rotamaks and spheromaks. Just as a matter of prefixes, what does toka-, roda-, and sphero-, allude to?
BELLAN: The tokamak was the original one. That's a Russian acronym, because the Russians invented the tokamak. Mak, I think was machine, and toka was toroidal in Russian, so it was like toroidal machine in Russian. Then, when people came up with these other things, they decided that mak—
ZIERLER: Stuck.
BELLAN: Spheromak was like a spherical one, because a tokamak is like a donut, it has a hole in the middle. A spheromak doesn't have a hole in the middle. Then a rotamak was something they came up with in Australia that involved a rotating magnetic field that—I couldn't understand that for a while too, and then I actually went to Australia, and spent a month with a group there, and got interested in that. The way they made the current was very different from a tokamak. A tokamak is like a transformer, where you have a primary and secondary, and you induce a current. In the rotamak, I'd say, the picture is more like you have angels descend from heaven with chariots, and each angel grabs an electron and carries the electron along in its chariot and then you get a current, because all these electrons are moving along. There's no primary or secondary thing. The rotamak works something like an induction-motor, like vacuum cleaners and record-players use that. You have a rotating magnetic field and that captures electrons that want to stay in a field line, and you cause the electrons to rotate around, and that way you get a current. It was a nifty idea. It's kind of died out, although it picked up a little bit in the FRC community. I guess there's a guy at Princeton who's still doing rotamak-type stuff, Sam Cohen, has been pushing that all those years. I did a little work on that.
ZIERLER: Are all three pursuing similar goals, or are they more different?
BELLAN: They were all intended to be fusion devices. I mean, the difference in effort in these things is orders of magnitude. The total amount of money that's gone into tokamak research since year zero is probably, I don't know, including ITER, probably 30 or 40 billion dollars. The total amount of money that's gone into spheromak research over the years from year zero is, I don't know, maybe 50 million dollars or something like that. The total amount of money that's gone into rotamak research over the years is maybe like 5 million dollars or something like that. It's just a few people working on it, and the parameters are totally non-competitive.
ZIERLER: Paul, I have images of shooting in my mind. Tell me about your work on spheromak plasma guns and spheromak injections into a tokamak.
BELLAN: The people used the term gun for making a spheromak. The analogy is to blowing bubbles. In fact, you could almost make a soap bubble system that looked like a spheromak. I don't know if I can describe it. But suppose you were blowing soap bubbles, but you decided to be ambitious, and you had something with two wands, one that was, say, five centimeters in diameter, and one that was twenty centimeters in diameter. Instead of putting the soap film on the small wand or the big wand, you put the soap film between the two wands, so you had an annulus of soap film. Then you blew up—you had somebody puffing up from underneath, so this annulus would start distending upwards, and then it broke off, and it would make a toroidal bubble. That turns out to be a little bit oversimplified, but for these purposes, that's not a terrible analogy. The gun is the equivalent of the two wands that's making something that initially is shooting up. That you can imagine that before it's breaking off, you'd get something that's distended and it would be long, like the jet pictures I showed you, and then it would break off and make this bubble. Before it breaks off, it's a jet coming off and you could call it a gun, because something's shooting out from it. Just like the astrophysical jet.
ZIERLER: Paul, moving into the 1990s, what were some of the questions you were pursuing in magnetohydrodynamics?
BELLAN: I don't know if I could remember well enough one decade from another. I think I was working on—then I was trying to inject spheromaks into tokamaks, I think this was a helicity injection. The idea was that the spheromak has a lot of helicity, and the tokamak needs helicity. If you can merge a spheromak with a tokamak, it would be like throwing batteries into the tokamak and have them spontaneously line up just the right way, to sustain the current in the tokamak. Then you could also refuel the tokamak that way, because the tokamak has fuel in it for the fusion and if it burns up, you need to put more fuel. If you could shoot a spheromak in that's got lots of deuterium and tritium that merges with the tokamak, then that would be useful. I was working on shooting spheromaks into tokamaks. We built a gadget that did that. I had a student go to a lab in Texas that had a medium-size tokamak, and we did some experiments on that. In fact, it was those experiments that made me decide to go back to do more fundamental stuff, because I didn't like the fact that we were just trying to push parameters and try to get something, get something, get something. We didn't really understand what was going on. We were just turning knobs higher. I decided that the field wasn't at the point where you could do that, that we had to understand things better. I built this machine where the whole goal was to understand what was going on.
ZIERLER: What about ion dynamics, what was some of your work in ion dynamics at that point?
BELLAN: You're probably talking about stochastic ion heating. We discussed that a little bit before. In our little tokamak, I was collaborating with Raul Stern, who was a generation older than me, who was an expert on laser-induced fluorescence. I was supervising a student who built up an LIF, under supervision of me and Raul. We saw the hot ions I mentioned last time. That involved ion dynamics, so I've been working on that on and off continuously. I mean, just recently we did a paper on that. I've always been interested in particle dynamics, particle orbits, and I'm still doing things that relate to that.
ZIERLER: The paper, Alfvén resonance reconsidered, you had worked on this previously. What were you reconsidering at that point?
BELLAN: According to MHD, magnetic field lines can behave like guitar strings. You can pluck them and you can get a resonance. There's a whole theory of what are called field line resonances. What I went through it, I decided I didn't believe it, and so I wrote a paper knocking that, and I guess made lots of enemies.
ZIERLER: What was the contention exactly, what were you seeing?
BELLAN: I claimed that what they were seeing wasn't field lines behaving like elastic bands, because the mathematics shows they don't behave that way if you looked at it carefully. There was singularity in the MHD equations that indicated that. But I felt if you went beyond the MHD equations, the singularity disappeared, and there was a more complicated thing going on that wasn't a field line resonance at all. I guess I wrote a paper claiming that what they were seeing was related to resonance cones, which was my favorite thing. I don't know. I had a few people agree with me, and lots of people disagree with me, and I haven't really kept too much track of what's going on since then.
ZIERLER: Paul, the Caltech compact torus injector, is that the spheromak you were referring to?
BELLAN: That's a spheromak. Compact torus is another name for spheromak.
ZIERLER: Why have two different names?
BELLAN: I think compact torus could encompass more than spheromaks. There was another device called an FRC, Tri Alpha Energy is working with FRCs, and compact torus included both spheromaks and FRCs. There were conferences where they had both of them and this was a way of keeping everybody happy by having a more general name.
ZIERLER: I asked you before about publishing in Physics of Fluids, then I see later on, you start publishing in Physics in Plasmas.
BELLAN: It's the same journal basically, they just—
ZIERLER: They just changed the name?
BELLAN: Yeah.
ZIERLER: What does that tell us that they changed the name from Physics of Fluids to Physics of Plasmas? Was plasma physics becoming more prominent at that point?
BELLAN: I think so. There was an editor, Ron Davidson, who I think was the first editor of Physics of Plasmas. I'm not sure whether it was his idea to do it, but the field had become big enough and separate enough from fluids that Physics of Fluids was really two journals in one at that point, so it made sense to split it into two.
Entree to Solar Physics
ZIERLER: Paul, when did you start to get interested in solar physics?
BELLAN: That came about from being at Caltech, I think. That's pretty circuitous. I guess I heard some talks by Hal Zirin, who was the Director of the Big Bear Solar Observatory. I had zero knowledge of solar physics. I had no idea that it involved plasma physics or anything. Then I saw some of his talks and I saw some pictures of that, and I didn't think much of that. Then, I guess a couple things happened. I'm piecing them together. One was, I was interested in electric arc furnaces, and I had an undergraduate work on that, and we saw some stuff that reminded me of the pictures of the sun there. I thought maybe there was some connection.
Then I was at a conference in Italy, in Lake Como, where there was a guy from General Atomics who had been working on yet another similar tokamak device, called a reversed field pinch, that's sort of kind intermediate between a tokamak and a spheromak. He proposed building something based on reversed field pinch technology to simulate solar coronal loops. But he came from a big wealthy lab, and so he was incapable of thinking small. His idea was to build a five million dollar solar loop simulator experiment that would be, if you think of a donut and you cut it in two to get something like a McDonald's arch, half a donut, then that would be his five million dollar solar arch experiment. He gave a talk on that, and of course he wasn't able to get the fusion people to pay for that, because it wasn't fusion, and he couldn't get the solar people to pay for it, because they didn't want to spend five million dollars in lab experiments. Around that time, I was just starting my spheromak experiments, and I had come up with cheap ways of making spheromaks. It dawned on me that I could probably make a solar loop cheaply too. I decided to try that, it was a bootleg thing.
ZIERLER: On what basis? Why would spheromaks be relevant for this?
BELLAN: I think I heard talks where people pointed out—these solar loops are like half of a spheromak also. Again, you take a donut and you cut it in half, not the way you normally cut it for putting it in a toaster, but the other way, if you're sharing it with somebody. You get an arch, and I heard people—it was sort of a motherhood statement where people were saying, "oh, spheromaks are similar to solar physics, it's the same equations,". I thought, well, if it's the same equations, maybe we can make a solar loop in the lab. I did a bootleg experiment in the 1990s I guess, that only marginally worked at first. We blew up something, and we didn't have a decent camera to take pictures of it. We just barely had a picture of it. The guy who was funding me from DoE was very nice, and when he saw that he said, "I'll give you money for a good camera, what do you want?" I told him and he gave me fifty thousand dollars to buy a good camera. Then I started taking really beautiful pictures of it and then I was off and running.
ZIERLER: I'm curious why at this relatively late stage in the game, you had come on to solar physics? Were there not obvious connections earlier?
BELLAN: No. I don't know. I mean, maybe there were, but you have to realize the literature in those days was very different. Because now we can look up anything we want on the web. There, you'd go to your conferences, and you'd see your buddies. I went to the plasma conference and there weren't any solar physicists there. Occasionally, I'd be at a workshop where they'd invite somebody from a different field to talk about it, so maybe occasionally there'd be a solar physicist. I would subscribe to Physics of Fluids or Physics of Plasmas and read that, but wasn't really aware of the existence of these other things. Now you just go on the web and you put in a key word and up spews a hundred things, but you had to go to the library and look in scientific citations, which was like an encyclopedia. You had to know what you were looking for; you didn't accidentally come across things. I just wasn't aware of these things.
ZIERLER: Finally, Paul, last question for today, in the 1990s, as computers were becoming more powerful and widely adopted in scientific laboratories, did that change your work at all?
BELLAN: Yeah, I think so. Sure. I mean, our experiments were computer controlled, because when I started there were no computers and you would have a motor moving something along and an XY recorder with a pen, maybe a digital meter you'd read off something and write numbers in a book. Then computers came, then you started writing things automatically and having multiple channels and you could collect orders of magnitude more data and control things much better. It was totally different.
ZIERLER: On that note, we'll pick up next time the early 2000s going forward.
[End of Recording]
ZIERLER: This is David Zierler, Director of the Caltech Heritage Project. It is Friday, April 15th, 2022. I'm delighted to be back with Professor Paul Bellan. Paul, it's great to be with you. Thank you for joining me again.
BELLAN: Thank you, I'm happy to be with you.
ZIERLER: Paul, today I'd like to start with your work in the early 2000s. When you were working on accretion disks and jets, was that your first foray closer to astrophysics or had you done work on this previously?
BELLAN: I think it was my first foray.
ZIERLER: What was going on at that point? What were some of the things happening in astrophysics where you were contributing?
BELLAN: I don't know. I was on the periphery. I think I had a postdoc at that time, Scott Hsu, and we were working on the jets. I guess it was at that time that we start to realize that we had jets. The experiment was intended to show how spheromaks formed, and I had no idea that there were jets, and that slowly became apparent. Slowly, like over two years or so, that we were making jets and that they were going unstable. I think Scott was the one who thought that this might have some connection to astrophysics, and this got me to start thinking about that. Started reading the astrophysical literature and realized that we were doing that. We made some contact with a colleague at JPL, David Meier, who was studying astrophysical jets, and who had, I think, a postdoc at the time from Japan. I don't remember his name right now, it'll come to me. This postdoc was doing numerical models of astrophysical jets and was seeing kink instabilities in them. His numerical models looked an awful lot like our experiment. To an outsider they looked more or less the same. That made me realize that this was a connection. I started reading up on it, and giving talks on it.
I also got asked to give talks on this at a conference on high-energy density laboratory astrophysics. There's a group at the Livermore Lab using the National Ignition Facility, their big laser, meant to do laser fusion. As a sideline, they were claiming that they were achieving astrophysically relevant parameters in their experiment, that they were getting very high densities or temperatures or whatever would be appropriate for certain astrophysical situations. They had set up a meeting held every second year on that. They invited me to give a talk, I think in Tucson, on my stuff. I didn't really fit under high-energy density. They had some kind of definition of what high-energy density was, and I was nowhere near that, but the astrophysics laboratory part was related, and they were interested in that. I got into that community. I was kind of off and running with astrophysics at that point, and I just slowly learned it from then on.
I found the whole thing rather amusing, because when I was a graduate student at Princeton, the Princeton plasma physics lab where I worked was organizationally an offshoot of their astrophysics department. It was related to Princeton University like JPL is related to Caltech, was an off-campus lab, so I knew a lot of people on it, but there was an organizational chart that showed the university and the astrophysics department. There was a little box underneath the astrophysics department, saying plasma physics lab, even though the plasma physics lab was probably 25 times as big as the astrophysics department.
But the courses I took were taught by people sometimes from astrophysics, and astrophysics students took the same courses, and I took courses in the astrophysics building. But I never actually took any real astrophysics courses, I just took plasma courses. In any case, when I got my PhD, it said, doctorate in astrophysical sciences, because that was the astrophysical part. I have a doctorate in astrophysical sciences, but I didn't know any astrophysics, so I felt I was making good on what it said in my degree. Now I really do know astrophysics, or at least some astrophysics.
ZIERLER: Paul, with your work at that point on accretion disks and jets, was their opportunity for internal collaboration at Caltech? I'm thinking, for example, of Roger Blandford, before he left for Stanford.
BELLAN: Yeah, we were talking to him. I wouldn't go so far as to say we were collaborating, but we did talk to him, and he was aware of our experiments. We got some pointers from him, and then over the years, I've had some modest contact with him. I think there was a bit of a ships passing in the night issue in that he's much more generalist, he was looking on many aspects of astrophysics and this was just one little point. I'd get all excited about the specific thing we were seeing, but that was just like one of a thousand things that he was interested in. It was a little bit hard to get him to focus on our particular experiment. He would be polite and listen, but he had a lot of other things on his mind also.
ZIERLER: Paul, I'm curious the extent to which it was rather late in the game for you to become involved in astrophysics. What does that tell us more generally about the broader aloofness of plasma physics from astrophysics?
BELLAN: I think things have changed in the last 20 years, or even more than that. Because I think a lot of this has to do with just how the world has changed, with the explosion of communication and information, more than with people. Before the worldwide web and desktop computers, when you just worked with printed things and books and journals in the mail, it was hard for you to go outside of your own field. You'd spend all your efforts in your own field and that was that. I suppose you might meet somebody in another field at a cocktail party and you could make a connection that way, but generally you wouldn't be exposed to things in other areas so much. I first became aware of it when I was doing some work on a kind of plasma wave called an Alfvén wave, and I just knew about it in my context. But the web bibliographic searches were just coming up, and I did a search on Alfvén waves and I discovered there were all sorts of magnetospheric applications which hadn't occurred to me. I looked into that and actually wrote one or two papers on that. I taught myself enough to say things about that.
I think this business of jumping from one area to another, or at least looking at how what you do applies to a different area, became easier once it was easier to access information. You didn't have to go to and sit in a library for four hours looking through the stacks to find the right thing. I think I was one of the early people to try to make this jump from laboratory plasma physics to solar- and astrophysics, and other people have done that a lot more since. It's more common now, but I think that it's just easier to communicate across fields now than it was before.
ZIERLER: Paul, your entrée through accretion disks and jets into astrophysics, what about that was happenstance and what about that is logical, that that would be the entry point for a plasma physicist to start thinking about astrophysics?
BELLAN: The accretion disks was something that came later, I would say. The jets, what we were doing in our experiment was making these jets and we had electrodes and capacitors and power supplies and coils that was the apparatus for making the jet, and then the jet would be in the plasma. I spent a lot of time wondering, what is the astrophysical equivalent of our electrodes and power supplies and gas-injectors and coils? I'm still worrying about that. That's got to be the accretion disk. There were theories about that, that seemed to explain it very quickly, and I used those also, but then when I thought about them, I felt that there was something wrong with those theories. They were being used out of context. In fact, this led me to a sort of favorite bugaboo, I think in a lot of areas people will use things out of context. They'll be some theory that's a great theory in its own context, but then people will take it and use it someplace where the assumptions that went into that theory don't hold. I find this very common. I think this was going on with accretion disks.
I guess you're touching a nerve. There was a theory that was very popular, and is very popular, in the astrophysical world, having to do with accretion disks. It's called the magneto-rotational instability. I think it came into being in the 90s, maybe the 80s. In astrophysics, there was a big problem having to do with angular momentum. When I first heard about this, I didn't understand it very well. My first feeling was who cares, and second of all I didn't understand it. Then over the years, I understood what it was and then I realized that it's a big problem and now it's intuitive to me. It went from being something I didn't understand at all to being intuitive. Anyhow, this problem has been kicked around in astrophysics for a long time and it has to do with accretion. That is, that when things accrete they come out with angular momentum, but as they go to smaller radius, their angular momentum would increase according to the basic laws, but it clearly doesn't and something has to get rid of the angular momentum.
People couldn't figure it out. This magneto-rotational instability was proposed and all the astrophysicists latched on to it. There were meetings with hundreds of people in awe listening to speakers about this and there's huge numbers of papers. I think there's over 1,500 papers on the magneto-rotational instability. I tried to understand it. It was a very complicated derivation, and I went through it and I could barely work through this simplest version of it. But it always struck me as being too contrived and I didn't really believe in it. I set about trying to come up with alternate explanations. I think I started this probably about 10 or 15 years ago, and so I've been hacking away at some kind of alternate to the magneto-rotational instability over all this time. It's been very interesting. I came up with ideas that I liked a lot. This was a theory effort that was motivated by experiments, that is, what's the equivalent of the power supply in our experiments. I published a bunch of different papers and right now a student of mine and I have a paper that's in press on that that's the latest version of that, and I really think we're coming up with good explanations that are excellent alternates to the magneto-rotational instability.
There was something I've probably mentioned before, I think, did I mention canonical angular momentum before?
ZIERLER: A little bit, but we should go into that some more.
BELLAN: I felt that this was a critical thing with accretion disks. That's something that comes straight out of classical mechanics, with Hamiltonians and Lagrangians. It's one of the first things you learn. But it seems to have gotten forgotten by the astrophysicists. I thought they knew about it. I wrote a bunch of papers on it, but when I did a Google search or a web, I guess, a bibliographic search on all the times that canonical angular momentum was mentioned in astrophysics, I only found my own papers, I didn't find anybody else's. It didn't seem to be there. But the general idea is that when you have a magnetic field, ordinary angular momentum that we're familiar with is not a conserved quantity. There's something else that's more complicated. It's ordinary angular momentum plus another term, that involves the magnetic field, that's conserved. Then when you look at it that way, you can come up with all sorts of explanations for what's going on in an accretion disk. I attacked that problem from many points of view, and I've been making good progress on it. I found accretion disks interesting from that point of view.
Then, the dusty plasma stuff turns out to be related to that too, because accretion disks become protoplanetary disks, and they have dust in them. The dust is charged, and so that's related to the dusty plasma stuff I've been doing. It's all interrelated. We can't make accretion disks in the lab. The lab has electrodes and power supplies, but I think that in astrophysics you need something to replace the lab metal electrodes and power supplies, and I think that's what the accretion disk is doing. The people who study accretion disks are generally different from the people who study jets, and so there isn't—
ZIERLER: Paul, why is that, why would there be that bifurcation?
BELLAN: Because I think astrophysics is really mainly observational. There's one group of people just looking at one thing, and another group of people looking at another thing. The theorists are separated from the observers, so everybody's kind of narrowly focused on their own little topic. There isn't a whole lot of integration in my opinion, between seemingly disparate topics. I think they know that accretion disks are related to jets, because they're always seen together. But I don't think there are people trying to study how an accretion disk drives a jet. Or if there are any, they're very, very few.
ZIERLER: Paul, in the past 20 years has there been a greater integration in the fields of plasma physics and astrophysics?
BELLAN: I think so, yeah.
ZIERLER: For the better? This is a good development?
BELLAN: Yeah, I think so. If you look at it historically, they almost started together and then they separated and now they're coming back together again a little bit. I mean, not tightly together, but there are a fair number of lab experiments that are trying to do astrophysically relative things, and I don't think that existed a generation ago.
ZIERLER: Paul, what remaining work needs to be done in this field and why should astrophysicists be alert to what plasma physicists can offer?
BELLAN: I'm not sure how to answer that. I don't think we understand lots of things. I guess, to answer your question, I'm thinking of something that Alfvén wrote. Alfvén is the great man of plasma physics. He was somewhat controversial at the time. In at least one of his books he said something that I kind of liked. He said that the astrophysicist tends to think of space as being filled up with objects, like stars and planets and maybe there's some stuff in between them, but they're all individual things. They're little spheres that are connected together. Alfvén was saying you should really think of space as being like electric circuits, that there's stuff flowing from one place to another that's interacting with something at a distance. It's a different point of view. He pointed out that before the space age, before there were the first spacecraft, that's how we thought about the solar system. There was the sun and there were the planets and the moons and that was that. Then when spacecraft went up, we discovered that there was the magnetosphere and the solar wind and all this stuff going on in interplanetary space, which was quite complicated. There were all these circuits of stuff flowing from one place to another and affecting things elsewhere. The heliopause and the magnetopause and the magnetotail and all this stuff, but you wouldn't know about any of that if you didn't have spacecraft going up and measuring it in situ, because you can't really see it from a distance.
He suggested that there's the same kind of stuff going on in astrophysics, but since you can't send spacecraft up there, you can't make local measurements since you don't know about it. In plasma physics, there's this tremendous hierarchy from large-scales to small-scales. It's like the earth we live on or the air we breathe, there's winds and then there's molecules and the molecules are made of atoms and there's all these levels of stuff, but plasmas have many levels of description, and we can only see the largest scales from a distance, but the small scales are important. We're seeing that in lab stuff in the magnetosphere. I don't think the astrophysicists have really caught up with the small-scale stuff, or the importance of the small-scale stuff.
ZIERLER: Paul, does this relate to cosmology at all, the large-scale structure of the universe?
BELLAN: I don't know. Probably not so much. It depends on how you define things, but I don't think of plasma physics as being related to cosmology particularly. Although, because it's a very classical field, there are people who talk about plasma concepts in a cosmological situation.
ZIERLER: I'm thinking in the early universe, for example.
BELLAN: There are probably things going on there that are like that, I'm just not familiar with them. Occasionally people use plasma concepts there, but the ideas are much woolier, at that point. People aren't dealing with these rather definitive things that we're dealing with in laboratory plasmas and space plasmas now.
ZIERLER: Paul, switching topics, a nomenclature question, helicity injection, what is that?
BELLAN: I just gave a lecture on helicities, so I'm all primed for that. You could probably ask me in my sleep, and I could still do it. There are two or three concepts I want to mention. There's something called a magnetic flux tube. You have magnetic field lines, and you have a bunch of them and they go round and then they join on themselves. You could have something like an automobile tire or bicycle inner-tube, with magnetic field going around in a circle. That would be a flux tube and the ends are connecting on themselves. Then you could imagine two flux tubes that are linked like links in a chain, you have something like that. The linkage of two flux tubes like that, that's helicity. It's also equivalent to a twisted magnetic field. It's mathematically the same thing. You can actually do parlor tricks illustrating helicity. I just did it in class a couple days ago, but I don't have anything right here that's like that. But if you take this and you put a twist in it and then you tape it to the ends, you've got a loop with one twist in it. I'm giving you homework.
ZIERLER: Yes.
BELLAN: Take a ribbon and make, I guess, two twists, I think is what you'd want to make. I can't actually remember what it is. One full twist, actually. One full twist. It comes out on the same side, when you go around to tape it up. Then once you make that, take a scissors and cut it lengthwise going along the length, all the way along. When you do that, you'll find that you get two things that are linked together. It seems like magic, it's like a magic trick. There's a way of counting up the twists and the linkages and you find that you can turn a twist into a linkage or a linkage into a twist, and either way it shows up that's helicity. You get helicity in a magnetic situation where you have a magnetic field in a plasma, and then you arrange for an electric current to flow along that magnetic field. From Ampere's Law, if you have a current, that won't make a magnetic field, but that new magnetic field will be around the current, and so now you've got two magnetic fields. The original straight one and then the one from the current that's going around the original one, so that's a linkage type situation. To inject helicity, you need to have a coil to make the original field, and then you have to have a current that flows along the magnetic field made by that coil. Then you're injecting helicity. This is what goes on in jets and spheromaks and tokamaks, they all have twisted magnetic fields and to make the twisted magnetic field you've got—twisting it up is equivalent to running a current along it, which is injecting a current.
ZIERLER: Paul, your work on solar plasmas and simulating them in laboratory experiments, how do you do that, what does that look like?
BELLAN: We make a system that starts with something that's essentially a horseshoe magnet. You've got a north pole here and a south pole here, and so you get magnetic field that goes from the north pole to the south pole. This is in a vacuum chamber. We also have some holes in the magnets where we can squirt gas through. We have fast gas valves that can inject some puffs of gas in there, so there's a cloud of gas in there. Then we apply high voltage between the north pole and the south pole, like 5,000 volts difference between the north pole and the south pole. That high voltage will break down the gas and we'll have plasma there. Then the magnetic field going from the north pole to the south pole acts somewhat like a wire. The particles can flow easily along the magnetic field, but they can't flow perpendicular to the field, so it acts like a wire that guides the current. The electric current will flow from the north pole to the south pole, because of the high voltage. Now we've injected helicity, because we've got a current flowing along the magnetic field, and the current will tend to pinch upon itself. It has a self-force that will tend to squeeze it down, so you'll get a narrow loop, and so you've made a solar loop.
ZIERLER: What is the broader application of this? When you simulate it, how might that be applied beyond the laboratory?
BELLAN: We're not trying to come up with an application that would be used in everyday life. The point to this is really to understand what's going on in the sun and to simulate things that are happening on the sun. We are able to see these loops expand and go unstable. I argued that these loops behave like two jets pointing at each other, and we were able to show that. We've shown that you can prevent them from expanding by adding an extra magnetic field to them. We've shown that they can expand in an explosive way by having them held down and then suddenly break loose. There are many aspects of this that we're able to study, but we're not trying to make a gadget that would be useful from this.
ZIERLER: In thinking about what's happening on the sun, I'm curious, do you consider heliophysics to be a subset of plasma physics?
BELLAN: No. Plasma physics—they're different regions of the sun, and the outermost region, basically the surface of the sun and then going out from the surface, is pretty much described by plasma physics, by magneto-hydro dynamics. It's very similar to the physics in our experiment, which is why we can do things relevant to the solar situation. But what I do is not relevant to the interior of the sun, where things are more of a, I wouldn't say solid, but they're not really plasma-like.
ZIERLER: Although, just observationally, we can't possibly know what's going on interior to the sun.
BELLAN: Actually people do know a lot about the sun. It's not my field, but they have things that are equivalent to seismographs, things that measure earthquakes, and they can measure sunquakes, and they can do it with spectacular resolution. They can do tomography on that, so they can actually see inside the sun. They can even see what's going on on the other side of the sun, they can look right through the sun with this. It's fairly spectacular. It's not my field, but there is a fair amount of knowledge of what's going on inside the sun.
ZIERLER: Paul, I'll ask you to translate a paper title, A Model for the Condensation of Dusty Plasma. What does that mean, what is a dusty plasma?
BELLAN: Normal plasma has electrons and ions in it, and the electrons are of course much lighter than the ions. In many plasmas, the electrons are hotter than the ions, although in some plasmas they're the same temperature. But there's a whole class of plasmas which are weakly ionized, where there's a lot of neutrals and not much plasma. In those plasmas, the neutrals are cold, the ions are in thermal equilibrium with the neutrals, and the electrons are hot. The electrons being hotter and lighter, go faster than the ions. If you stick a material object, like a speck of dust, in a plasma like that, it will be impacted by the neutrals, the ions, and the electrons. If it's hit by the neutrals, nothing much happens, it's just like a speck of dust in the air we're breathing. If it's hit by an ion, the ion could stick and leave a positive charge. If it's hit by an electron, the electron could stick and it would have a negative charge. But because the electrons are going much faster, the flux of electrons onto the little speck of dust is much more than the flux of ions, so the dust grain will tend to become negatively charged. As it becomes more negatively charged, it will start repelling electrons, because it's negative and they're negative. Things of the same sign repel each other, and maybe ions will come in a little bit easier, because ions are attracted to that. This will go on until it gets to an equilibrium where there's equal numbers of electrons and ions hitting it, but it'll remain negative, because the original electron flux was much bigger.
Now, you've got this dust grain, which is negative, sitting in a plasma. But if you think of what a plasma is, it's a collection of negative and positive charged particles. The dust grain is a charged particle, it's just very big and heavy, but it's a charged particle. Your plasma is now a collection of two types of negative particles, electrons and these dust grains, which are negative. They could have different amounts of charge on them, depending how big they are and what the plasma is doing. Now if you have a lot of dust grains, you could have a situation where the dust grains start repelling each other, because they're all negative. If you have lots and lots of dust grains, so they're close together, then let's say you have three dust grains, one in here, one in the middle, and one on this side. If the one in the middle tries to go to the left, it'll get repelled. If it goes to the right, it'll get repelled. It'll try to stay in the middle. If you have lots of dust grains, every dust grain will try to stay in the middle, relative to the other dust grains, then you'll wind up with a lattice situation, a crystal. That would be considered the dust grains condensing into a crystal lattice.
This was first observed I think in the 1990s by people in Germany, and it's commonly seen in dusty plasmas. You get something like solid-state physics all over again, but with these dust grains forming a crystal structure. The neat thing about it is that it's almost on a human-scale. The dust grains can have varying sizes, a few microns to even bigger than that, and the spacing between them could be hundreds of microns, so you can see this with ordinary cameras. You don't have to buy terribly fancy equipment, just a Nikon camera like you'd buy in a camera store, with the appropriate lens, can see these lattices. The paper I think you're referring to was trying to calculate the circumstances under which this lattice would form, because you don't always get a lattice, you have to have enough dust grains crowded together so that they're repelling each other to form this lattice.
ZIERLER: Paul, when you're looking at plasma flow that's both dynamic and stagnating, is that the same plasma flow? In other words, is it the same plasma that's either moving, it's kinetic, or it's not? Or is it two categories, two separate categories of plasma?
BELLAN: I'm not sure if I understand the question. I'll try to answer what I think you're getting at. I mean, plasma is a bunch of particles. You can characterize the particles different ways. You could talk about the average velocity of all the particles. Say that you've got a trillion particles and they're going all different directions, but if you average their velocity, you find that the average velocity is a hundred meters per second. We do that with the air we're breathing. When you talk about the wind speed, that's the average speed of all the molecules in the air. But if you look at an individual molecule, it's not going at the average speed, it's going at some random velocity that's probably much bigger than the average speed. The average of the square of the random velocity is what we call the temperature, or at least it's related to that. A plasma could have its average velocity moving and so that would be a dynamic motion of the plasma, or it could not have its average velocity moving, so it would be a static plasma.
When you talk about stagnation, that's a term where you've got a flow, let's say a water-hose pointing at a wall, and when it hits the wall it can't have a flow, because the wall is standing still, so the velocity has to go to zero. The technical term is that the flow has stagnated at that point, it's gone to zero. Then the flow would usually go at sideways, at that point. But interesting things will happen. Or if you have two hoses pointed at each other, then right at the middle they can't be going to the left or the right, because they're equal and opposite. That would be a stagnation point, and that would be an interesting thing, where things often are a little bit different.
ZIERLER: Paul, when you started to work on spheromak formation, was that a basic science kind of approach or were you thinking specifically about getting closer to nuclear fusion?
BELLAN: I think I started on it from a fusion point of view, a little bit. I think I started on it really because I thought it was kind of neat physics and it had a potential for fusion. But I was probably more attracted by the neat physics aspect. I had this idea that people should work on what they're good at and what they're interested in, and if everybody does that, then there'll be the most useful output. I don't think it's a good idea for somebody to work on something just because they think it's important. I think they're better off working on what they're good at, and then more will get done. Or what they're interested in.
ZIERLER: Then where do flux tubes enter the picture when you're working on spheromak formation?
BELLAN: Spheromaks are kind of flux tubes already. A flux tube is like a hose carrying a magnetic field. If you bend it on itself, then you've got a torus, and so that would be a twisted—and spheromaks have helicity, so you have a twisted flux tube bent on itself. An everyday example of that would be like a smoke ring. If somebody blows a smoke ring, the magnetic field in a spheromak is very much like the magnetic field in a smoke ring. That's a toroidal structure. A smoke ring doesn't have a twist in it, but if you added a twist to it, then it would be similar.
Dusty Plasma Problems
ZIERLER: How did you get involved in night-shining clouds, what was the point of entrée there?
BELLAN: That's a dusty plasma problem. Those clouds, they're called noctilucent clouds, and I guess when you start studying dusty plasmas, then people mention them a lot as a natural example of a dusty plasma. I did some reading on that. I wrote a paper, which I don't completely believe in right now. I think there's some aspects I still believe in, but I don't think it's entirely correct, that addressed an issue in noctilucent clouds. I'm trying to remember it now, this is quite a while ago. People were bouncing radars off of noctilucent clouds. Noctilucent clouds are kind of curious tings, they exist in polar regions, like very far north, or very far south, on the earth. If you're in Sweden you can see them. They exist in summertime mainly, and at very high and very precise altitudes, like between 82 and 85 kilometers altitude. They're only a couple kilometers in extent, and they're always about the same height. They don't change much, so it's like somebody planted something at a certain height, and it's just there. It's very cold at those altitudes. It's about 150 kelvin, so it's more like an astrophysics type temperature than the temperature we're used to. Of course, the pressure is very low there, it's more like a lab experiment. It's about a hundred thousand times lower than the pressure at sea level. It's at the edge of space basically. If you're a little higher then you say you're in orbit and if you're a little lower than you say you're in the atmosphere. It's kind of at where the atmosphere turns into space.
These clouds can be seen with the naked eye, if you're in polar regions. They come and go. They're not ordinary clouds, they're little ice grains and they're charged much just like I described charged ice grains. There's a whole group of people who have been studying this for many years. There are radars on the ground that will try to reflect off of them. In principle, our radars shouldn't reflect off of them at all, because they're tiny bits of dust that are a few nanometers in size and the radar wavelengths are meters, and a meter radar shouldn't be at all sensitive to that. But they do see fairly substantial reflection, so I was trying to come up with a scheme for that. The scheme's coming back to me now. There's another thing around there that's also kind of strange. There's something called the sodium layer, have you heard of that at all?
ZIERLER: No.
BELLAN: Have you heard of—I forget what it's called now. With telescopes looking at the stars, there's a problem that the earth's atmosphere moves around and you get blurring and twinkling and you can't see very well. That's why people want to put telescopes in space. People came up with the idea that you could do a trick that would allow you to cancel out the effect of the earth's atmosphere and basically see just as well as you could in space, or at least almost as well. This trick, it's called adaptive optics. It's now being used in all modern telescopes. There are various versions of it, but one version goes like this. At also around 85 kilometers high, there's a thin layer of sodium atoms that seems to be coming from continuous flux of meteoroids, micro-meteoroids, coming from outer space, that ablate and they the sodium melts at that layer and you wind up with this layer of sodium. Then you can shine a laser from earth up in the sky and tune it to resonate with sodium atoms and so it'll go up to 85 kilometers and cause the sodium to fluoresce. You focus the laser so that you get a dot in the sky. You've made an artificial star effectively. Then the artificial star will twinkle and move around, and you measure that, and you can figure out what the atmosphere is doing. Then if you're clever, you can arrange your telescope to undo that and you get back a sharp picture. This works pretty well. There's a solar telescope that has been doing this big time at Big Bear and they're able to see things down with a resolution of about 60 miles or so on the sun now, which is fairly impressive.
It turns out that the sodium layer interacts with the noctilucent cloud layer, and the noctilucent clouds eat up the sodium. There's a depletion of the sodium when there's noctilucent clouds. I learned from my reading that when sodium is deposited in ice at these cold temperatures, it doesn't start burning, which is what would happen in a lab. If you put sodium in water it bursts into flame, but at these cold temperatures it doesn't do that, and it actually forms a metallic coating on the ice. I had this idea that the ice grains get coated with metal and that's why they would reflect the radar so well. But then there was some other problem with it that made me think maybe that's not what's going on. I went to a meeting on noctilucent clouds in Stockholm and I saw the noctilucent clouds and I met people in that community and became aware of that issue. There was a curious thing about noctilucent clouds, at least one guy has proposed something which I think is pretty bizarre. It's a natural phenomenon, it's a cloud, and he wrote a paper claiming that these things didn't exist before 1885. It's like saying tornadoes didn't exist before a certain time.
ZIERLER: On what basis can one make that claim?
BELLAN: He claimed that noctilucent clouds got instigated by the Krakatoa volcano explosion in that year, and that we're still seeing the remnants of that and before that there wasn't anything. His argument was that there's no historical record of noctilucent clouds before Krakatoa because something you could see with the naked eye and if you take something like the aurora, there are Chinese manuscripts from 2,000 years ago talking about seeing the aurora. He looked through all the ancient manuscripts and nobody had any mention of these things and so he had other arguments claiming. He made a reasonably decent case for that, and it's conceivable that he's right.
ZIERLER: Are these clouds so specialized that they don't really tell us anything else about cloud formation or other clouds, or can you make those extrapolations?
BELLAN: No, you can't. They're really quite different from ordinary clouds.
ZIERLER: Is there a concern with global warming that we might lose them?
BELLAN: No, but they've been changing over the years and there's a concern that that means something or other, but I'm not sure that there's a consensus on what it means. Because there are people who spend their lives measuring these things, and they have seen some trends, and I guess at some point somebody called them the canary in the coal mine that was warning us about some terrible thing that's going to happen, but I forget what it was.
ZIERLER: I wonder if you can explain your interest in Hamiltonian dynamics as it relates to plasma physics.
BELLAN: Hamiltonian dynamics is kind of the first thing you learn in physics. It's just a glorified Newton's law, another way of expressing F=ma, but in fancier ways. It's what everybody thinks of when you think of physics, how things move and accelerate and have orbits and trajectories. But the beauty of Hamiltonian mechanics is that it exploits symmetries. When you talk about angular momentum—you've heard of conservation of angular momentum?
ZIERLER: Sure.
BELLAN: But if I asked you to explain why angular momentum is conserved, you probably couldn't. That used to bother me, because when my teachers would tell me—then explain something because of conservation of angular momentum, that never struck me as a particularly good explanation, because I didn't see why angular momentum should be conserved. The answer is, you can't really see why it should be conserved. It sort of comes out of the mathematics of Hamiltonians, and it's related to Newton's ideas that something will keep on going on its way unless there's something opposing it. But the Hamiltonian says that in a stronger way. It says that if a coordinate is ignorable, which means that it can move in the direction of that coordinate and nothing changes, then the momentum associated with that coordinate is conserved. Something is symmetric about an angle, then the Hamiltonian tells you that the momentum associated with the angle, the angular momentum, is conserved. I don't think you could really see it intuitively; it just comes out of the mathematics and it's just the way the world is. But all these equations, like Schrödinger's equation and Newton's equations, are kind of postulates that explain how the world works, but they can't be proven, you can't start from something simpler in saying there's this simpler thing that we agree upon that then shows that Newton's equations are correct. It's just something that seems to work.
ZIERLER: Paul, tell me about the experiment where you used high-speed cameras to look at plasma jets, and you saw that they were, if I understand correctly, torn apart?
BELLAN: I think we've discussed that already a bit, but we had this camera that I bought I guess 18 years ago now, that was about the fastest thing you could get at the time, and it still is. There really haven't been any enormous improvements in that area.
ZIERLER: Is it commercially available, is it specific for science?
BELLAN: It's commercially available. It's not specific for science. I think it was intended more for studying artillery shells. If you look at the websites advertising it, they'll show you an artillery shell being followed or exploding. I think these cameras have their antecedents in a different kind of camera that was used to study atom bomb explosions in 1945. The idea was to have a camera that could follow things that took place in a microsecond, so you needed a shutter speed that was faster than a microsecond. The original cameras did this mechanically. They were pushing the limits of what you could do mechanically. They had a mirror, if it rotates around fast, then an image will move real fast. If I have a piece of film far away, I can have the image move in the field real fast. If I rotate the mirror as fast as I can, I can just barely make something that could capture things in a million frames per second.
But then in the digital age, people figured out how to get rid of all the mechanical stuff and make digital cameras. Digital cameras actually can't work that fast, but they use image intensifiers, that's like what people use for night vision googles, and they have an electrical switch on them to turn the night vision google on or off. That's electrical, and you can do that real fast. If you put that in front of your digital camera, then you can get a very fast shutter speed. Then if you want to make a movie camera, there's a good \ practical way of making movie camera, if you don't care about the money. That is, you buy a separate camera for each frame of your movie. That costs lots of money, but then you got a movie camera. You do that and you fire the camera sequentially and now you've got that. This camera I have works like that, except clearly you can't afford to buy too many cameras, so it has a limited number of frames. It basically has eight frames, but then it has a trick where it's able to get sixteen frames. Then it can operate real fast. It's shutter-speed is five nanoseconds, five millionths of a second. It can, in principle, take movies at the rate of 200 million frames per second, which is actually faster than what we need.
ZIERLER: Did you use this to look at solar flares?
BELLAN: We use it for both our solar experiment and for our jet experiment. They're similar. The thing is, our experiments take place on a time-scale of—at least the duration for the solar experiment is about five or seven microseconds, and the jet experiment, in one mode, is also about five or seven microseconds, and in another mode, it's about forty microseconds. Then things happen during that time, so you want to be able to look from one microsecond to the next, or even a fraction of a microsecond, maybe a tenth of a microsecond, to the next. Having a camera this fast is very good, because we can study things that are going on that fast with it.
ZIERLER: What were you learning about solar flares, what was interesting about this project?
BELLAN: We've seen a bunch of things. We've seen how they expand. My students came up with something a few years ago that was pretty neat. I had argued that there are jets coming from what are called the footpoints. Remember, I described the north and the south pole, so there's a jet coming from the north pole and a jet coming from the south pole, and there's plasma flowing up and that's what fills the coronal loop. You were asking about flux tubes. The magnetic field going from the north pole to the south pole, that's the flux tube. At least, think of a tube of field lines, a bundle of field lines, going from the north pole to the south pole, that would be the flux tube. If there's flows of plasma up then these magnetic field lines get filled up with plasma. There are magnetic forces that cause this whole thing to expand, so that the sort of arch rises up.
What my students saw was—we'd seen years ago that the top of this thing often would have a dip in it, and we'd assumed that that was a projection of a helix or a kink on it. But it didn't really make sense, because it would always look the same. If it was a projection of something, it should be random, it should sometimes be one way, and sometimes another way, but it was always the same way. Then they realized that it wasn't a projection of something, instead there are these jets coming up and they would collide at the top, and the top would have a higher density than the rest, because they were smashing into each other and building up there. Since there were forces accelerating things up, at the top it would be heavier, because there was more mass there because of this collision. If you have the same force and more mass, the acceleration is less, because the acceleration is the force divided by the mass. The top would not keep up with the rest and so when you looked at this thing expanding, the top would be dragging behind and so it would look like a dip. We saw that with our cameras. We also played games with multiple colors. We would, to show that there were jets, we'd use one gas coming from the north pole, and one gas coming from the south pole. Say nitrogen on one and hydrogen on the other. Take pictures, put filters in the camera and color-code things so we could distinguish one gas from another. You could see a red plasma hitting a green plasma. That was something we were able to establish.
ZIERLER: Paul, I'm curious if you have any opinions on the idea that solar flares affect climate on earth? Specifically, there are some people who think that part of the warming earth issue is increased solar flare activity.
BELLAN: It's possible. It would be a very indirect route, because the solar activity does affect the earth's magnetosphere and ionosphere, we're talking about a hundred miles up. But the problem is that the densities of things up there are maybe a hundred thousand or a million times less than they are on earth, so I guess if you're talking about, say, affecting a person, it's like you pull one hair on a person and saying that's going to affect their life span. It seems a little bit unlikely. There just isn't much there. But it may have some effect in that it could affect clouds at high altitudes. For example, like the noctilucent clouds are at an altitude where solar activity conceivably could affect them or it could affect the earth's magnetic field, which could indirectly affect polar regions and aurora. There's some tiny things that might happen, but it's not obvious that there'd be some major direct hit.
There are some things, though, that are major direct hits. The solar physicists like to make a big fuss about this. I'll give the historical example of this. There are two historical examples. The most recent one was, in 1989 there was a solar storm that knocked out the electric power in the province of Quebec in Canada for about a day and caused many tens of millions, maybe hundreds of millions of dollars, worth of damage. The issue is the earth's magnetosphere has electrons going around in a circle. This is somewhat related to the Van Allen radiation belt, but these electrons are doing lots of things, and one of the things they're doing is moving in a circle around the earth. It's not like an orbit, because they're making different kinds of orbits, but they're stuck up there and they're sort of drifting around in a circle. This circular motion of the electrons constitutes an electric current, that's called the ring current. Although each electron is tiny, there's lots of them, and the amount of current in the ring current is a big current. I forget what the number is, but it's a lot.
When there's a solar storm, it can hit the earth's magnetosphere and interfere with that ring current and interrupt it. If you know about electric transformers, a transformer has a primary and a secondary, and so interrupting the ring current is like changing the current in the primary of an electric transformer. If you do that, that will make a voltage on the secondary or change the current in the secondary, but the secondary will feel something. Changing the ring current was like changing the primary in a transformer, and that induced currents on the earth and things that could conduct it, and the things that could conduct it were electric power grids. It sent spurious currents along long-distance electric power grids. It turned out the current isn't that much, but the current was a very low-frequency current, so that was effectively like a DC current. That got added to the normal current of the transformers in the power grid and they don't like to have a DC current on them. They're meant for AC and if you have a DC on it, that will affect the transformers in the system. It'll wreck them. It's sort of like having a see-saw that's delicately balanced to go back and forth, and then you put a big weight on one end and then doesn't work properly. It burned out the transformers, they blew up, a whole string of transformers blew up. This could happen again. It would be if you had a big solar flare, it could knock out electric power grids. Electric companies are aware of this.
At the dawn of solar physics, I guess 1859, there was a famous incident. This is sort of in the book of Genesis for solar physicists. There was a British scientist named Lord Carrington, who built the first serious device for looking at the sun. He had some kind of lens or mirror or something that would make an image of the sun on a table. When he just got it working, there was a solar flare, and he had an assistant with him, so they both saw it. It was a big solar flare, in fact, it's the biggest solar flare that anybody has seen since 1859, which is quite amazing because he just set this up and he sees the big one. That's called the Carrington flare, and the earth, at least the world wasn't industrialized the way it is now, but they did have some things. They had telegraph wires all over the place, and this Carrington flare was enough to cause the wires in the telegraphs to have high voltage on them to spark and to cause fires, so there were fires around the world because of the Carrington flare. Anyways, people could in retrospect figure out how big the Carrington flare was, and it was much bigger than the Quebec one, and if it happened now, it would do billions of dollars of damage to our economy. It would knock out things all over the place, so solar physicists are warning about that.
ZIERLER: We have no effective defenses as of yet?
BELLAN: We have some defenses. One defense we have is that, you have some warning of it, because when it comes from the sun, it doesn't come instantly. It takes about 24 hours, 24 to 48 hours, to make its way from the sun. It's not the radiation, it's actually a plasma, somewhat like my experiment in spheromaks. That is, ejected from the sun and traveling towards the earth, and it takes a day or two to hit the earth, and then it knocks the daylights out of the earth's magnetosphere and can cause all this. You can have warning and then if you know it's coming, you can tell people to batten down the hatches, disconnect this and turn off that. A flare like that might be very harmful to astronauts in space too, because there'd be a lot of X-rays associated with it. Those would come more or less instantly, so you wouldn't be able to protect yourself against that. You wouldn't know it was coming.
ZIERLER: Paul, tell me about your work with solar loops and what it's impact was for space weather science.
BELLAN: I'm not sure whether we've had an impact yet. We've been working with the solar physicists over time in understanding how these loops go unstable. I think we've had some impact I guess. We've done some experiments where we had two of these loops interacting with each other. The people in the sun have sometimes seen that and at least one or two have referred to our work. I think we've had some moderate impact in how people think about things.
ZIERLER: More generally, in space weather science, are they primarily plasma physicists?
BELLAN: Yes, space weather is essentially plasma physics.
ZIERLER: How far back does that field go, space weather?
BELLAN: I think it goes back to the second World War, maybe earlier. That's kind of the beginnings of plasma physics, because it had to do with short-wave radio propagation. Did you ever listen to a short-wave radio or you know what short-wave radio is?
ZIERLER: I know what it is, yeah.
BELLAN: So, ham-radio operators and international broadcasters have used short-wave radio, so you can listen to Radio Moscow or the BBC or whatever or Radio Australia, sitting in Pasadena, you have a radio that would listen to those things and the question is, how did that work? It worked by radio waves coming from a transmitter and reflecting off the earth's ionosphere. It would be like a mirror. It would bounce back and forth between the ionosphere, which is maybe hundred or a few hundred miles up, and the ground. You'd get what's called a skip, so it might take two or three skips to make its way there. When this happened, people were surprised. There is a book about Marconi that I read a while ago called Thunderstruck, excellent book, that described this. When Marconi got radio going, everybody thought it would be line of sight, and they were very surprised that you could do this long-distance stuff, and it was because of the bouncing off of the plasma in the ionosphere. That kind of instigated one form of plasma physics, to understand the ionosphere, which is a plasma.
Then the solar storms kind of upsets the ionosphere. Short-wave radio is notoriously flaky. It's like the weather. Sometimes it works, sometimes it doesn't work. There could be a good day when you can hear BBC perfectly like it's coming from next door, and then another day you can't hear anything at all, or you can hear it and it's fading in and fading out. This had military implications, because if you're trying to communicate with your army that's hundreds of miles away, or your airplanes or whatever, and it's fading in and out, this is a big deal. It's still a big deal. The military got interested in this, and funded people to study this. Since the sun is making the storms that affect this, you have to study solar physics. The radio weather will be affected by the sun spot cycle. When there's more sun spots, there's more of these solar storms and the radio is affected more.
There was a professor at Caltech, Hal Zirin, who was a solar physicist. He certainly was aware of this history, and he explained to me that how the Americans, and British, and Germans were all studying the sun during the war because they were trying to understand how the radio communications would be. It was like meteorology basically. Even recently, I remember hearing that when the US was in Afghanistan 15 years ago, and there was a helicopter landing on some ridge, and people were trying to communicate with the helicopter, there was some kind of solar activity that interrupted the communications and caused some trouble there. Now, it affects spacecraft, because spacecraft are in orbit and they feel a little bit of friction from whatever plasma is up there. If the plasma gets disturbed, then the friction changes and their orbit changes and so they jump to a different orbit, and you can actually lose a spacecraft. They don't even know where it is, and you have to spend some time for that. Or, these things can destroy a spacecraft. People don't like to talk about it, because when it's a military one, they don't like to talk about it, and when it's a civilian one, they don't want to say, oops, we lost our spacecraft. But there have been numerous examples of spacecraft destroyed by these things.
ZIERLER: I want to ask about how the Caltech plasma jet experiment got started, but first, MHD simulation. What is that, what is MHD?
BELLAN: MHD stands for magneto-hydrodynamics. There's two ways of looking at it or at least two ways of coming at it. One way of coming at it, is from ordinary hydrodynamics, where you talk about things like fluid flow and pressure. If you have a pressure gradient that'll make a flow, your water hose you have high pressure at one end and it makes the water flow. Down there, are meteorology, the winds of high-pressure area and low-pressure area that when air goes from high-pressure to low-pressure, you study all that that's hydrodynamics. Then if you have a plasma, then there's the additional feature that there can be electric currents flowing, because the plasma is conducting. The electric currents will make magnetic fields, and now you have electric currents and magnetic fields, and when you have electric currents and magnetic fields, you get forces. The product of the currents and the magnetic field in a vector sense, gives you a force. If you add that, now you've got both the pressure pushing things around, and the magnetic force pushing things around. If you have an electro-magnet and a magnet, they'll attract each other, so that's a magnetic force. That can act on the fluid. Then the plasma has the interesting feature, which I think I mentioned before, that it behaves like a super-conductor, so that the magnetic field is actually frozen into the plasma. If the plasma moves the magnetic field lines move with it, and so you have this sort of puzzle where you've got currents and magnetic fields and plasma. The magnetic field can push the plasma around, but the plasma carries the magnetic field with it, as it's pushed around. The equations that describe that are magneto-hydrodynamics.
ZIERLER: The experiment itself, how did that get started?
BELLAN: I'm not sure what you mean by how it got started.
ZIERLER: What was the funding, what were the science questions that motivated you to start the experiment?
BELLAN: The solar experiment—I'd been working on spheromaks, which involve magneto-hydrodynamics and the magnetic helicity and all these things we've been talking about, and I attended a conference in Italy, where a guy spoke about building a device that would simulate a solar coronal loop. It hadn't occurred to me really that it was similar to the spheromaks, but it was kind of like a half a spheromak. If you take a circle and you cut it in two—I don't know if I got something that's a circle—but if you cut it in two, not the way you cut a bagel, but perpendicular to that, and you get an arch. A solar loop is effectively like a half a spheromak, but that way.
This guy was proposing to use, not spheromak technology, but something closely related that I think we discussed, reverse field technology to make a loop. It was going to be a very expensive experiment. Then I had come up with a scheme for making spheromaks cheaply and I thought maybe I could make a solar loop cheaply that way. I did it as a bootleg experiment, and it sort of worked. I was getting funding from Department of Energy then, and the guy who was funding me was a really nice guy. He used to be kind of hard on me, but he was nice. We saw this. I had a camera that was a high-speed camera, but it didn't work very well. It wasn't a movie camera, it was just a still camera. It took rather terrible pictures. They were like old newspaper pictures, where you could hardly see anything. They were just black or white, there was no grey. It was like black or white and nothing else. I complained that I couldn't take decent pictures with this, but it looked like I was seeing some kind of loop. He said, "well, what are you really wanting?" I said, well, I'd love this super-expensive camera. He said, "write me a proposal for that." I did that and he gave me the money and I bought this very expensive camera and then we started taking pictures that really looked really good. The whole thing seemed worthwhile and kind of took off from there.
ZIERLER: What were some of the findings?
BELLAN: We saw we could make the loops and that they would expand and that we could inhibit their expansion. Basically, all this stuff that we've done recently. Take multicolor pictures. Most recently, we're seeing various instabilities in X-rays coming out of these things.
ZIERLER: Paul, your work with water ice grains in laboratory plasma, was that related at all to your previous work in the clouds?
BELLAN: Yes.
ZIERLER: In what way?
BELLAN: It's almost the same thing. The ice grains in the clouds are little grains of ice that are electrically charged in a background that's cold neutral gas, and the experiment is basically that. The ice grains in the experiment seem to be a lot larger than the ones in the noctilucent clouds, and not quite sure why that is, but that actually makes it easier to study them. But it's certainly a related thing.
ZIERLER: Another nomenclature term, Rayleigh-Taylor instability, what is that?
BELLAN: That's something you actually would know, you just didn't describe it by that term. If you, say, take a glass and you put water in it and you put oil in it, so that the water is, say, heavier than the oil, and you somehow arrange it so the water is on top of the oil, so you have a heavy thing on top of a light thing, then you know that's unstable. You learn that when you're two years old. But they can't just trade places, because there's no room for them to trade places. However, what happens is, an instability develops where ripples develop at the interface between the two of them, and they trade places. I'm sure you've seen things like that. There are gift items you can buy, where they have two types of fluid, one red and one blue, and you put one on top of the other and then they form ripples and trade places. It's a heavy fluid trying to trade places with a light fluid, but doing it by ripples. I think when you pour wine from a wine bottle, it gurgles, that's a Rayleigh-Taylor instability, because the wine can't just come out of the bottle as a whole. You can't have the wine trade places with the air all in one shot, doesn't work that way, so it makes little ripples and trades places that way.
Plasma and NASA
ZIERLER: Paul, your work in 2016 and I think maybe early 2017, where you were looking at determining the wavelength of energy flowing through plasma in space, that was subsequently applied in a NASA mission, tell me about the determination. What had you discovered?
BELLAN: That was something that actually was successful. I mean, not that the other things weren't successful, but this had a strange history. I had written a paper about something else, and I put in a little thing at the end on that, about some sort of corollary that we might use this for something else. I hadn't thought much about it, and then somebody at Goddard picked up on it and contacted me, and they wanted to actually use this. I think I tried to get it to work, and it didn't quite work. Then I discovered that there was a rather minor mistake in it, which I corrected, and then it seemed to work. I did a computer calculation where I simulated the kind of data that a spacecraft would measure, and I showed this thing would work with that. Then I gave that technique to the Goddard people, and they used it and it worked very well.
The issue is that suppose you've got a wave and it could be a sound wave or a radio wave or a light wave. There are plasma waves that are different from all of those, but waves have certain things that make it a wave. There's a wavelength and they'll be a frequency and they'll be a direction. We usually don't work with the wavelength, we work with the inverse of the wavelength, which is called k, and that's a vector, and k has a direction. It could be in the X direction or the Y direction or the Z direction, so it's a vector. It's related to the wavelength. The question is, how do you measure k, which is like how do you measure the wavelength of something. The way you measure length of things, is you get a ruler. If I ask you to measure the length of you shoe, you'd put your shoe beside the ruler and you'd look at one end of the shoe and you'd see 0 on the ruler, and you'd look at the other end of the shoe and you'd see 11 on the ruler, and you'd say your shoe is 11 inches long. You have to make a measurement at two places.
The question is, if I gave you a shoe and I told you to measure the length of the shoe with a measurement at only one place, could you do that? Could you do that with a wave? Could you measure the wavelength of a wave with just a single point measurement? This scheme I came up with allowed you to do that. It has to do with Ampere's Law and a limiting form of Ampere's Law that is actually appropriate for a great many waves that people are interested in. Basically waves which are different from light waves. The big thing that Maxwell did was to introduce something called displacement current, and that's what allows you to have radio waves and light waves. But if you go back before Maxwell, there was Ampere. If you didn't know about displacement currents and you didn't have this term, and the equation mathematically was curl of B is equal to J. Whereas with Maxwell, it was curl of B equals J plus displacement current. For lots of waves, it turns out that the original pre-Maxwell form is okay. You don't need displacement current. That form of Ampere's Law, it's a vector equation, but mathematically I'll simplify it, it's something like k times B is equal to J. This is oversimplified, but it just gives the idea. If you could measure J and you could measure B, then you could figure out what k is. That's the general idea. B is the magnetic field and J is the current density.
I didn't think spacecraft were capable of measuring current density, because that means you've got to measure all the electrons coming and all the ions coming and take the difference between the two and then do this in the X direction and the Y direction and the Z direction, and do it fast, so you're doing it for a high-frequency wave. That seemed like a tall order. But apparently my friends at Goddard told me they could do that. They could measure J that way. B is easy to measure, that's no problem. They did that and they could calculate a k that way, using my method. As I said, it's a little bit more complicated than what I said, but I gave you the general idea. Then they also had the equivalent of the ruler. That's something you do at a single point, you measure B and J at a single point, and from that you could get k, and that's like getting the wavelength of the wave. But they also had four spacecraft, so that's like having the ruler, so they could check it by measuring it the conventional way, by effectively putting a ruler in and measuring it and they found that it gave the same result. It worked. Of course, it's easier and cheaper to use one spacecraft than four spacecraft, so they went on to use that and they're continuing to use that in other situations I've seen. That was something that actually worked.
ZIERLER: How did NASA initially get wind of this?
BELLAN: I think it was a person at NASA who read my paper and contacted me and said they'd like to use this method. I think the paths of all these things is always rather circuitous, but the guy who—I'm not sure how he found it. The paper was kind of a diatribe about something else. There was something somebody had done which I didn't like the way they did it. Actually, that paper was an interesting paper because I purposely chose the most boring sounding title for it. Usually people do the opposite, but I had my reasons.
ZIERLER: Paul, does your sense that the application of this for the MMS mission was more on a capability side or more on a basic science side?
BELLAN: I think it does both, actually. Certainly basic science, but I think it improved the capability.
ZIERLER: In what way? What does it make possible?
BELLAN: I think they found that if they used it on the four spacecraft and averaged over the four spacecraft and made that like one big spacecraft, then I think they got better results than the method they were using before. It was more precise. There was something better about it. Then it does mean that in situations where you only have one spacecraft, you could measure the wavelength of the wave, whereas otherwise you wouldn't be able to. It gives you a capability. These spacecraft cost—MMS probably costs about a billion dollars, so one spacecraft was maybe a quarter billion dollars. Roughly speaking, this method would save you 750 million dollars, if you want to exaggerate. But you could use one spacecraft instead of four.
ZIERLER: I've asked some general questions about how you've gotten into related fields from plasma physics. What about AMO physics, atomic, molecular, and optical physics? Was the opportunity there through the accretion disks in astrophysics, or was it the other way around, you were already doing AMO techniques?
BELLAN: I'm not sure if I would say I'm doing AMO. We've been doing laser induced fluorescence, which I suppose falls under that. But I think people often lumped plasma physics into AMO, but I think that's more of an organizational thing than a real physics related thing. Atomic and molecular and optical basically means it's not plasma, it's not ionized. To the extent that I'm doing spectroscopy, it would fall under that. Maybe we use things that would be listed as AMO as tools of what we're doing, but we're not pushing the frontiers of AMO particularly.
ZIERLER: More broadly Paul, I'm curious, with all of the emphasis on sustainability research at Caltech, do you see a role for plasma physics?
BELLAN: Fusion physics is essentially sustainability physics. That's how I got into the field when I was a student, I was interested in that, and it's form of nuclear power. I can give you the spiel about fusion. I don't know, are you familiar with the spiel about fusion?
ZIERLER: Oh, it's the hope for our future. It's unlimited energy.
BELLAN: The numbers are stupendous. There's enough fuel in seawater to power civilization forever. A gram of fuel would power a household for a year or ten years or something like that, I forget the numbers. It would be certainly a panacea. It has some hazards, but it's much, much less dangerous than fission physics, than fission reactors, because with a fission reactor you have like five years' worth of fuel sitting in a reactor all the time. If it blows up, you've got this five years' worth of fuel that gets spewed across the countryside. Then you've got lots of waste that can be turned into weapons. Fusion reactors have a little bit of that, I'm not saying they have nothing. They make the reactor itself radioactive, but they only have a tiny amount of fuel in the reactor at any time. You don't have five years' worth of fuel, you just have that second's worth of fuel. It's like your car engine doesn't blow up, because it doesn't have much gasoline in it at a time. Maybe the fuel tank could blow up, but the engine doesn't have much in it. It doesn't produce things that would go into atom bombs. You could if you wanted to, but it doesn't necessarily do that. The main problem with fusion is it doesn't work. It's hard to make and probably going to be expensive. But if you could do it, it would be great. That would be the ultimate sustainable thing. I worked on it for many years, but I guess I got a little bit bored with that and moved to these other things that were related. I may still occasionally do something that's related to fusion, but it's a long-term thing.
ZIERLER: I was wondering specifically with regard to the Resnick Institute, if there's an opportunity there because of all of the ways they're promoting sustainability in science?
BELLAN: There could be, I don't know, I just haven't pushed through that angle too much. I probably could. I'm pretty nervous about hyping things, I guess. I don't like making promises. I don't like saying, I'm going to develop this thing that's going to solve the world's problems. I find if you do that, you can get support right away, but then there comes a day when—
ZIERLER: "Whaddya got?" [laughs]
BELLAN: Yeah, people ask what do you have, and I don't want to get into that situation if I don't have to. I'd rather stick to not over-promising anything and just following my nose and trying to come up with interesting things.
ZIERLER: Paul, more recently, what has been some of your work with solar coronas?
BELLAN: What have we done lately? The most recent stuff we published was seeing Rayleigh-Taylor instability in a solar corona loop. Now we're looking at some of the twisting, the microscopic twisting, that goes on. In both the solar work and the jet work, the current emphasis is on the sequence of things that happen when one of these things goes unstable. This is probably somewhat related to solar flares. I mean, there are various pictures or analogues that I have. We were talking about flux tubes and the flux tube is twisted magnetic field lines and there's current flowing along. The mental picture I have is that's something like a rope, and the rope has strands in it, and the strands are wrapped around each other. It's braided. On the sun I think there could be a fractal arrangement, where if you think of a rope that, say, has five braids in it, but then if you look at one of those braids, it has five smaller braids on it. Then if you look at one of those smaller braids, it has five even smaller braids in it, and you keep on going down and down and down, and so at each level it's braided, but each braid contributes to the next size braid. We're not quite seeing that in the lab, we don't have the resolution for that. We have through one set of braids, but I think that's probably what's going on in the sun.
Imagine you have a rope and let's say you're picking up a heavy item with it. You've got a crane and it's picking up a truck or something with the rope, and the rope is rated at say 10,000 pounds tensile strength, and unfortunately the truck you have is 10,001 pounds. You pick it up and your rope isn't too happy. The rope doesn't break all at once, maybe one strand breaks. If you're standing near that, you might hear a pop when it breaks. Then if it breaks, now you've got one less strand. You're putting more stress on the other strands, because instead of sharing evenly between a certain number of strands, it's sharing between slightly less strands. Now there's more stress on the remaining strands, so another one pops. I think we're seeing stuff like that in our experiment, where the equivalent of having too much weight on it is passing too much current through it. You can imagine a wire that's rated at 100 amps, and you put 500 amps through it, and maybe there's some weak spot, that's like the rope breaking. Your wire could blow up there, like a fuse blowing up. We're seeing this happen in a localized place, it's where the weakness is. This is where we're seeing a Rayleigh-Taylor instability that's kind of like the thing where the rope is starting to be stretched and getting a little bit thin, and then the snapping. There are various things that maybe are equivalent to the snapping that you'd hear. We have waves coming off and we're seeing X-rays coming off and energetic particles coming off. You can imagine if you snap something, maybe a little piece of dust is flicked off at high-speed and hits you in the cheek and you're hurt from that. That's like a particle being energized and maybe hit something and makes an X-ray. We're seeing all this stuff going on and we're trying to piece it all together and see what's leading to what and how we can explain it.
This sort of thing is going on in a solar flare. Where if you ask somebody, what a solar flare is, they don't give you a very straight answer. They just say, well, it's, or this and that. It turns out that it's lots of things, that it's an explosion where many different things are happening. That there's a brightening, there's X-rays coming off, there's energetic particles coming off, there's this, that, everything you can think of is basically happening. It's like, if you set off a bomb, what happens? There's a sound wave and there's a blast wave and then there's a hole in the pavement and a flash of light. There are all these things happening and we're trying to piece them all together.
ZIERLER: Paul, we talked about ice grains in laboratory plasma, in clouds, what about interstellar ice grains? How do we know that there are ice grains in the interstellar medium?
BELLAN: People see that. There's actually two astrophysical places where there's ice and they're interrelated. One is the interstellar medium and the other is proto-planetary disks, that's sort of the precursors of the solar system. One is the spaces between stars and the other is like the pre-solar system around stars. I'm fairly aware of that, because I'm working on things related to that now. People can see that basically spectroscopically, either by emission or absorption. I think, interstellar stuff I'm not so familiar with, but that probably is emission. Could be absorption. But if you have ice, the ice is made of water molecules, and the water molecules have a temperature, and that means they're moving around and vibrating. If they're moving around and vibrating, they will emit electromagnetic radiation. Just like, I guess, anything you see that's emitting light. Shoots off photons and they have the characteristic photons that come off of certain wavelengths. Molecules can emit radiation, and it's done in the infrared. If you look through a telescope at the sky and then you take the light from the telescope and stick it in a spectrometer, you'll see certain wavelengths that you can identify as being water or ice wavelengths. They can, not just see that's water or ice, but they can see the phase of the ice. There's interest in whether ice is crystalline, that's the kind of ice that we're familiar with, or if you get a really low temperature, the phase of ice changes and it becomes what's called amorphous ice, more like a glassy ice. They can tell that spectroscopically.
Then with proto-planetary disks, I think they can see emission the way I described, but more commonly they see absorption. They'll look at a disk and they'll be a star, a distant star that's many light years away behind the disk. You look at the light from the star coming through the disk and the disk will be more or less transparent, but it's not perfectly transparent, it's a little bit opaque and it absorbs light from the star. If there's ice in the disk, it'll absorb certain wavelength of the starlight, so you'll see a dip in the starlight intensity at certain wavelengths. You can identify that with constituents, and you can also figure out that you've got ice and say something about the ice.
ZIERLER: Paul, on a personal level, when the pandemic hit and you had to shut the lab down, what projects needed to be back-burnered and what could you do remotely?
BELLAN: Well, we were building a new ice dusty plasma experiment, and I think I had spent about a year designing the thing and ordering the parts. The parts had finally all come in, and we had just started the assembly. Then the pandemic hit, and so the assembly pretty much stopped for six months. Then I had a student doing that, and then he could come in a little bit and he slowly put it together. We put it together, but we lost at least a year of time, I would say on that. Then the other experiments just stopped. People couldn't go into the lab for six months. I had a new postdoc who came, and he actually learned how to use the equipment without anybody physically there. It was all done by cellphones and Zoom. It's as if I taught you how to fly an airplane by yourself. It certainly slowed us down.
Then I tried to compensate for it by doing more theoretical and design work at home, so I did write one paper at home that I probably wouldn't have written, or at least it wouldn't have come out so fast, if I hadn't been stuck at home. This was something on ice dust. It was on why ice dust is elongated. I had some ideas kicking around for years on that, but I decided to put some more effort into it, and turned it into a real paper. Then one of my students had an idea. I had given a talk on something that was related to accretion disks, and I was thinking about how something could be done, and he said he was taking a class that would maybe enable this to be done. We worked on it, and this was all done by Zoom and no in-person thing, and it was all numerical calculation on the computers. That was extremely successful. We have a paper that's coming out right now on that. So, we kept busy.
ZIERLER: Paul, just to bring our conversation right up to the present, is your lab back up to its pre-pandemic level yet?
BELLAN: Almost. People are wearing masks, but they're working there. I'm still working at home a lot. I'm not in the lab as much as I was before. I guess I got used to working at home. I personally don't push the buttons on things in the lab or turn the knobs, I let the students do that. My job is more to suggest to them what to do or to interpret what they've done or help them when something's broken. Those sorts of things don't really require my physical presence so much, although it's somewhat better if I'm there. I've been coming in more and more recently, but I'm still at home and I kind of set myself up to do all my computer-type stuff at home, so if I go back to work, then I have to do a big change to get back there.
ZIERLER: It's kind of the new reality now.
BELLAN: I don't know. I think if things continue to die down, I think we'll get back to normal but I'm sort of afraid that things might flare up again. I'm not very interested in going to conferences right now. My students have been going to some, but I normally would have travelled to several conferences a year. People are starting to have conferences, but I'm really not very interested in that at this point.
ZIERLER: Paul, now that we've worked up to the present, for the last part of our talk, I'd like to ask a few broadly retrospective questions about your career, and then we'll end looking to the future. First, if you could survey the broad contributions in plasma physics over the course of your career, what have they answered beyond the specifics of the field itself?
BELLAN: I think people understand plasmas a lot better than they did when I started. I'm operating in the areas of plasma physics that we've discussed. There are some other areas that I don't work on that we haven't touched, but they have been influenced by the stuff that I'm working on. There's a whole field of low-energy plasma physics which has got enormous industrial use. All the chips in your cellphones. There's a shortage of chips in the world and you can't buy a new car because they don't have chips, so you ask how do they make the chips? They make chips using plasmas and there's a whole world of plasma physics there. There's a multibillion-dollar industry. Some of the stuff I do has some slight connection to that, the dusty plasma stuff has some slight connection to that. But I think people understand these plasmas a lot better than they did before. I think people avoided working with those plasmas for a long time because they're very messy. They don't have the elegance of the magneto-hydrodynamics. It's more like getting into the messy real world of stuff, not the elegant things that I find physicists like. People understood that a lot, and there's lots of money in it, so I think that's progressed a lot. Then in the fusion world, I think people understand the relevant plasmas quite well. They're tweaking things now, but I think that's understood quite well. Astrophysics I think is still a bit of a frontier. I think there's lots that can still be done there.
ZIERLER: Paul, for you personally, what do you see as your most significant contributions in basic science?
BELLAN: I guess the stochastic ion heating was something that I think was an important contribution over the years. That was something that started, I guess, almost 40 years ago now. I think we discussed that, but that showed how you could get a breakdown of what is normally considered invariant or constant of the motion. That could be important, and I think that's used a lot, or at least it's understood to be an important phenomenon that can give you a kind of heating in certain situations. Then some of the wave stuff I think has been important. Then I think we've made some impact with our jets and solar stuff that people paid some attention to that. I think because of the lab experiments, we had real hands-on explanations of what's going on. It's not something I can explain in a minute. There are different levels of explanation. The question is how long is somebody willing to listen to you? If I could spend an hour with you on the jets and draw pictures, you could walk away and have a pretty good understanding of what's going on. I don't think people had that sort of explanation before. I guess I always like to have an explanation for what's going on that you can understand in a hand-waving sort of way, and I think a lot of papers don't have that. They're just mathematics or they're observations, but there isn't this hands-on explanation. I think you should be able to do that in much of plasma physics, it's a classical field, it's not like quantum mechanics where you're introducing concepts that don't make any intuitive sense. This, in the end, should be intuitive, and so you should be able to explain it in an intuitive way, and if you can't, then there's probably something wrong.
The Application of Plasma Physics
ZIERLER: Paul, what about on the applied side? Where have you seen the most impact in applications, in translating the science?
BELLAN: In plasma physics? There's plasma thrusters. People have made rocket engines that are used in spacecraft now. They're used for making minor adjustments in spacecraft orbit or to change the direction the spacecraft is pointing. There also have been a few spacecraft where the plasma thrusters were used to propel the whole spacecraft. That's not common, but the plasma attitude correction stuff I think is common. That's an important contribution of plasmas. As I said, the making of all the chips that we have, is something where an application or a world wouldn't exist if you didn't have plasmas doing that. There are odds and end type things, like treating surfaces of materials with plasmas. I'm not sure if I can answer this question so well. There are colleagues of mine who specialize in drawing long laundry lists of plasma applications and telling you how you can't move for two seconds without being affected by a plasma, but I don't have that at the tip of my tongue. There are such things. There are things which are really plasma, and people don't think about it.
At one point, I was interested in electric arc furnaces that are used in the steel industry. When you get tired of your car and it starts leaking oil and it costs too much to repair, and you decide to junk it, it goes to a junkyard. They don't just throw it away, but they melt it down and make steel. They make steel and make a new car out of it. How do they do that? They have a big vat that they put scrap steel in and they have an electrode, where they draw an arc and heat it up and melt it. I used to think that this arc was melting it through the heat of the arc, but then I realized that it's actually a jet. A little bit like the jets that I have in my experiment. When the jet hits the steel, like when we were talking about stagnation, the jet hits the metal and it stops and all the kinetic energy of the jet gets turned into heating the metal and melts the metal. It's actually somewhat related to our plasma jets. The parameters are a little bit similar, except in our experiment we do it for microseconds, where they do it steady state. We have a hundred million watts, but it's just for ten microseconds. Where they have a hundred million watts day in and day out. I was interested in that a long time ago, and I had a patent related to that, and visited a steel mill, and tried to get the steel companies interested in a way of stabilizing these arcs.
Anyway, all the steel we have, maybe not all the steel, but I think over 50% of the steel that's used in the country comes from electric arc furnaces. That's an application of plasmas. There's not a whole lot of research going on, because it's a mature field. There are many various things like that, where there's plasmas. There are people who are trying to use plasmas for medical purposes now. You could have plasma torches that will cut skin and do it in a way better than a scalpel, or there's a whole group of people doing that sort of thing. Or kill cancer cells. I mean, you name it, somebody's trying to do something or other with it. I have not really ventured into all these far-out things, but I'm aware that you can draw a long list of them and show that plasma is somewhat ubiquitous.
ZIERLER: Finally Paul, looking to the future, for your students, as they're looking to chart their career, what's the frontier in plasma physics and how will that influence what you want to accomplish for however long you want to remain active?
BELLAN: I think the frontier now, in my mind, at least what we're working on, is to look at the interaction between different scales, where you have different kinds of physics. There's the large-scale physics and there's a lot of small-scale physics and there's some kind of grey area in-between, where the large-scale is driving the small-scale and the small-scale is driving the large-scale. That's a more complicated situation where you can't just break down a simple equation and say, we're going to ignore the small-scale and just talk about the large-scale, or vice versa. It's almost like an electrical circuit, where you've got a battery and a wire and a resister. If you were an old-style physicist, you'd just worry about a wire and you'd just say, oh, there's a current flowing in the wire and that's it.
Another guy might just talk about resisters, resisters heat up and doesn't know about wires, he knows about resisters. Then somebody else is studying batteries and doesn't know about wires. But if you wonder how does the wire affect the battery and how does the battery affect the resister, they're all kind of coupled together. I think that's the frontier from my point of view, because if you didn't have that, say if you looked at the solar flares for example, if you just had one model, then the thing would just sit there and it would do the same thing over and over again. But if it blows up at a certain point, it's like the story of the rope breaking. You put on too much force for the tensile strength and then you want to study why does it break, you have to start understanding tensile strength and how the molecules hold the rope together and how they detach. But that affects the thing breaking, so you've got to look at multiple scales. That part I think is interesting and we're doing that.
Then I think also there are sort of unknown unknowns, as was said by Donald Rumsfeld I guess. I'm not saying I'm a fan of him, but—
ZIERLER: The phrase has stuck.
BELLAN: The phrase has stuck, yeah. I think just not having a plan, but keeping your eyes open for new opportunities is very important. Trying to see things in a different way, or looking for unexpected things, is very important. I think that more progress is made that way, by seizing new opportunities and exploiting them, then by having some 20 step plan that you methodically follow through for 10 years. I don't think that works.
ZIERLER: That's something you can always be confident of, there will always be the new opportunities.
BELLAN: I think so. I think also you have to keep your eyes on what's going on in other fields, because, even though you might not be so interested in other fields, there could be a technique developed in another field that you can apply to what you're doing. Or there could be a device developed. I mean, say right now the cameras we're using can take pictures as fast as five nanoseconds per shutter-speed. But maybe there's some other field where somebody's come up with something that does it at one picosecond or something like that. If you don't pay attention to what's going on, then you wouldn't know about it. But if you pay attention, you discover, hey, this exists, and you could bring it from another field and use it. Conversely, hopefully plasma physics does something once in a while that would be useful to other fields too. I think one of the things I'm doing is continually bringing in technologies from other fields to diagnose our plasmas. When I started, I didn't know much about spectroscopy or about X-rays or about mass spectroscopy, or high-speed electronics, and had to learn all these things and bring them in. I think there continually will be new things that one can learn and bring in.
ZIERLER: Paul, it's been a great pleasure spending this time with you. I'm so happy we were able to capture all of your perspectives and insights. I'd like to thank you so much for doing this.
BELLAN: Thank you very much.
[END]
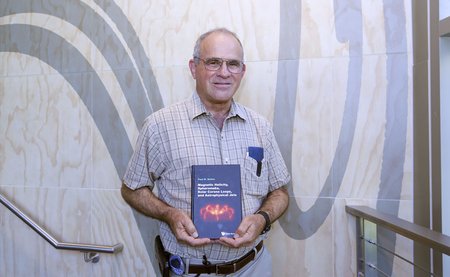
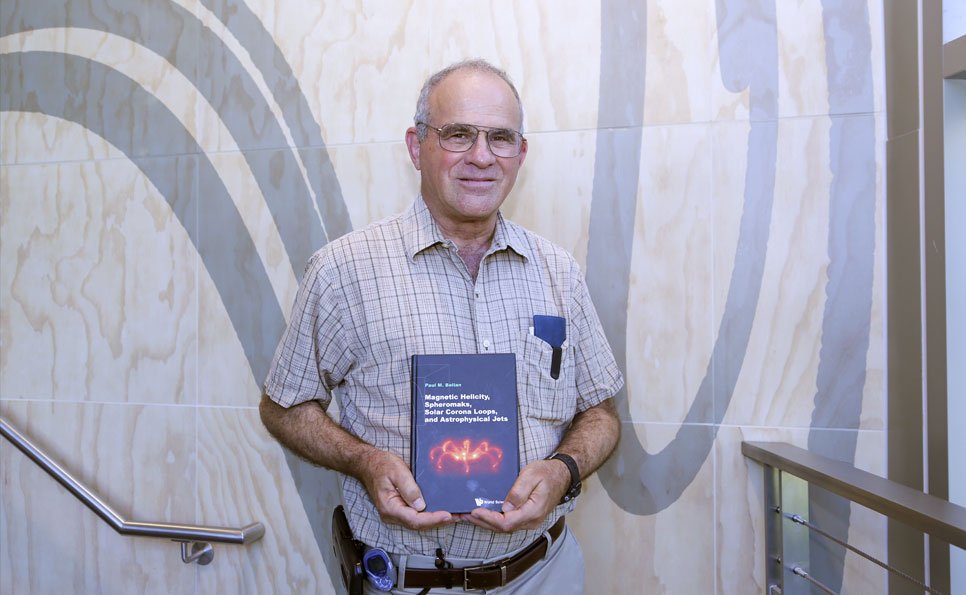